Van Phuc Nguyen,1 Josh Zhe,1 Umayr Ahmed,1 Justin Hu,1 Chi Hwan Lee,2-5* Yannis M. Paulus1,6* - 1 Department of Ophthalmology and Visual Sciences, University of Michigan, Ann Arbor, MI; 2 Weldon School of Biomedical Engineering, Purdue University, West Lafayette, IN; 3 School of Mechanical Engineering, Purdue University, West Lafayette, IN; 4 Department of Materials Engineering, Purdue University, West Lafayette, IN; 5 Birck Nanotechnology Center, Purdue University, West Lafayette, IN; 6 Department of Biomedical Engineering, University of Michigan, Ann Arbor, MI. *Corresponding authors. Email: [email protected] (Y.M.P.); [email protected] (C.H.L.)
Abstract
Vision impairment and blindness are critical problems worldwide. Major causes of vision loss include age-related macular degeneration (AMD), diabetes, retinitis pigmentosa (RP), and glaucoma. In order to improve treatment outcomes, multimodal imaging using photoacoustic microscopy (PAM), optical coherence tomography (OCT), fundus photography, and fluorescence imaging-guided treatment approaches can be utilized. This review explores novel nanopharmacotherapy treatments for a variety of ocular diseases. In the first section, the use of silicon nanoneedle-embedded contact lenses for the treatment of ocular angiogenesis including corneal neovascularization (CNV) is investigated. Next, a combination of chemotherapy and photothermal therapy with gold nanoparticles as drug delivery agents for the treatment of ocular tumors is discussed. Limitations and future applications are addressed.
Introduction
Ocular diseases cause hundreds of millions of people to experience vision loss and blindness. In 2020, more than 596 million people worldwide had been diagnosed with vision impairment, and its incidence is expected to increase to 895 million by 2050.1 The development and progression of several ocular diseases such as AMD, RP, and glaucoma are still not fully understood, although there is evidence demonstrating that retinal pigment epithelial (RPE) or photoreceptor degeneration, ocular inflammation, development of angiogenesis, or accumulation of metabolic waste can be hallmarks of these diseases.2 To date, many treatment methods have been investigated for these diseases.3 To remove corneal angiogenesis, therapies include anti-vascular endothelial growth factor (VEGF) topical therapy2 and conventional laser photocoagulation,4 but they both carry suboptimal efficacy and significant side effects.
To visualize the effectiveness of these treatments, multimodal imaging can be used. Optical coherence tomography (OCT) is a non-invasive technique that produces cross-sectional images of the retina. Retinal damage such as RPE death and neovascularization can be readily visualized with OCT.5,6,7,8 Photoacoustic imaging is non-invasive and uses non-ionizing laser pulses. Chromophores like hemoglobin, melanin, and lipids absorb the laser light, generating acoustic waves.9 Using various contrast agents, photoacoustic microscopy (PAM) imaging can provide high-resolution and high-depth images of the retinal vasculature as well as molecular or cellular imaging with contrast agents. PAM imaging has a high spatial and temporal resolution that allows for the differentiation between choroidal and retinal vessels.10-16 Contrast agents such as nanoparticles, nanorods, and nanostars have all been used to facilitate molecular and cellular imaging as well as provide improved visualization of the retinal and choroidal vasculature.9,17-19 This review will focus on gold nanoparticles as a potential contrast agent due to its versatility as a drug carrier, thermal agent for photothermal treatments, and biocompatibility.
Silicon Nanoneedle Embedded Contact Lens for Long-Term Drug Delivery
Current routes of drug delivery for ocular targets rely heavily on eye drops or frequent intravitreal injections. The former has a low bioavailability due to the many ocular barriers surrounding the eye. Only around 5% of the drug will reach the desired target, making eye drops cost-inefficient due to the large amount of drug lost during administration.20 The latter, while more efficient in delivering drugs, is off-putting for many patients scared of needles, is invasive, and carries significant risks including risk of infection that can lead to blindness.
Recently, nanomedicine has been explored as a novel method to improve the capability of drug delivery to the target.21 Nanomedicine can be utilized for various applications in biomedicine such as treatment, diagnosis, opening of the blood-brain barrier, and stimulation of neural tissues.21-23 Nanomedicine covers nanoparticles, nanoliposomes, nanocapsules, nanocages, nanohydrogels, nanomicelles, nanodendrimers, nanomaterials, nanobiosensors, and nanophotosensitizer probes.9,17,24,25 Today, nanomaterials with different sizes, shapes, and materials are being widely investigated for enhancing the current paths of drug delivery, improving the overall bioavailability of drugs, decreasing side effects and toxicities, and improving treatment efficiency.
Nanoneedles can be used for the delivery of drugs and other agents into the nucleus or cytoplasm in living cells. Nanoneedles can effectively transfect siRNA in corneal endothelium cells.26 Esfandyarpour et al. used a nanoneedle biosensor as an electronic probe to measure the electrical response of nucleic acids and proteins.27 Maurini et al. has used porous silicon nanoneedles to develop a nanoinjection approach for RNA interference therapy targeting the human corneal endothelium to effectively reduce levels of p16 protein and promote cell proliferation.28 In addition, their studies demonstrated that nanoinjection targeting the endothelial layer of explanted human corneas preserves the cellular structure and did not induce any significant apoptosis to the human corneal endothelium. To be effectively applied in different applications, the surface of nanoneedles needs to be modified by conjugation with other drugs and materials.
Park et al. has introduced silicon nanoneedles (Si NNs) integrated in a tear-soluble contact lens that can be effective, painless, and easy to administer to treat corneal angiogenesis. The Si NNs are first transferred to a polymethyl meth-acrylate (PMMA) film and then pressed into a contact lens-shaped mold to finally transfer the Si NNs to the tear-soluble lens. Park et al. reported a yield of >98% for the entire transfer process of the Si NNs. The final product had a lens thickness of 37-43 µm and a needle base diameter of 900 nm.24 A great benefit is that these Si NNs can be fabricated with different sizes and shapes. The Si NNs can safely penetrate ocular barriers without scarring the cornea as well as allow long-term sustained ocular delivery of drugs.24 Microneedles can also be used for drug delivery but are larger, which can lead to more discomfort and damage.29-32
Dissolution Kinetics
Park et al. reported quick dissolution of the water-soluble contact lens within a minute of application with multi-month-long sustained delivery of drugs through the Si NN (Figure 1A, C). It was also reported that no corneal punctures were found due to the NN, and the NN was inserted stably into the cornea without any washing away (Figure 1B, D). The drug release kinetics of the Si NNs could also be increased through the deposition of a passivation layer such as Al2 O3. Figure 1E-H shows the drug delivery process. Si NNs are able to degrade via hydrolysis reaction in the presence of tears. It was reported that the degradation of the Si NNs increases linearly with time from 3.5 nm/day to 16.6 nm/day. However, this reaction could be significantly slowed with a passivation layer of 0.05 nm/day. Once the passivation layer completely dissolved, the rate returned to the original degradation rate.
In Vivo Evaluation
Park et al.’s Si NNs were evaluated in a rabbit choroidal neovascularization (CNV) model (Figure 2). In order to monitor treatment efficacy, the Si NNs were used to release ocular drugs like bevacizumab to treat CNV.27 New Zealand white rabbits were used in this study. Rabbit eyes have been heavily used as the most translatable animal model for ocular diseases due to its many similarities to human eyes.33 Some of these similarities include corneal thickness, axial length, curvature, and even CNV pathology.34 Rabbit CNV development was monitored over 28 days with OCT, color fundus, and red-free imaging. Two experimental groups were tested: short Si NNs (10 µm) with 1.5 µg bevacizumab and long Si NNs (60 µm) with 14 µg bevacizumab. Three control groups were also tested: no treatment, subconjunctival bevacizumab injections without Si NNs, and non-dosed Si NNs. Both experimental groups proved to be highly effective in treating CNV without significant differences. Reduction of CNV was observed through the first 9 days with complete resolution of CNV within the 28 days. This contrasts with the no treatment group which showed no decrease in CNV throughout the 28 days. The subconjunctival injection group only showed an incomplete reduction of CNV. Most importantly, it was noted that the minimum dosage of bevacizumab required for CNV treatment (1.5 µg) was significantly lower in the experimental groups than traditional treatment methods such as subconjunctival injections or topical ophthalmic drops.35-37
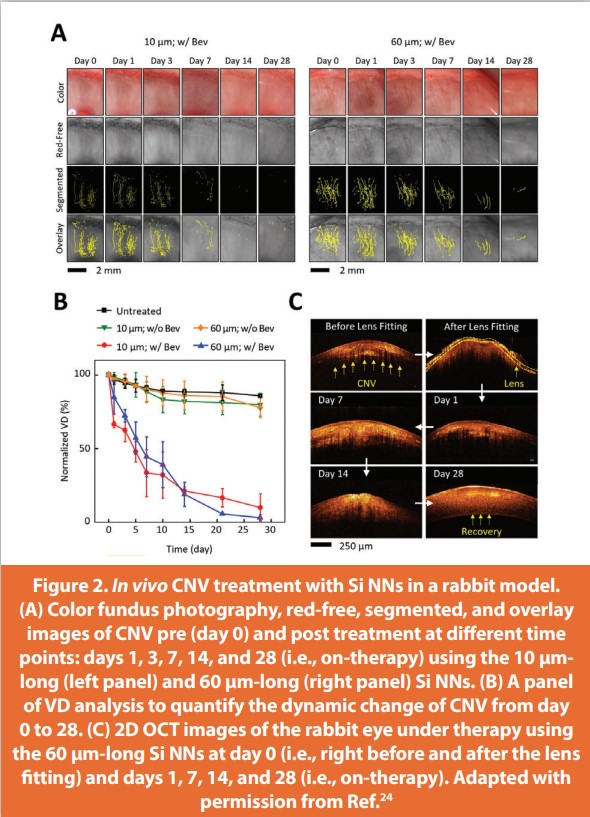
Combination of Chemotherapy and Photothermal Therapy for Treatment of Ocular Tumors
Common types of ocular tumors include metastases from other malignant cancers, choroidal melanomas, retinoblastoma, medulloepithelioma, retinal astrocytic hamartomas, neuroblastoma, leukemia, and infantile hemangioma.38,39 The diagnosis of ocular tumors is usually associated with very invasive methods such as histological analysis, lumbar puncture, and bone marrow biopsies. These methods are commonly performed in patients in clinics with a high risk of extraocular diseases.
Current treatments for choroidal melanomas have significant limitations, including high recurrence rates and infection.40 Using gold nanoparticles as a photothermal sink for tumor treatment seems highly promising and doubles as a contrast agent that allows for precise 3D visualization of the tumor’s position in the eye. Gold nanoparticles (NPs) readily absorb light, and the resulting heat can irreversibly damage tumors (Figure 3). Furthermore, drugs such as Doxorubicin (Dox) can also be administered using functionalized gold NPs. Dox has known chemotherapeutic effects such as inhibiting nucleic acid synthesis. Fucoidan (Fu) has also been recently investigated as a cancer medication given its anti-tumor and anti-inflammation properties.41,42 In addition, Fu is able to reduce the cytotoxic effects of heavy metal nanoparticles.43,44
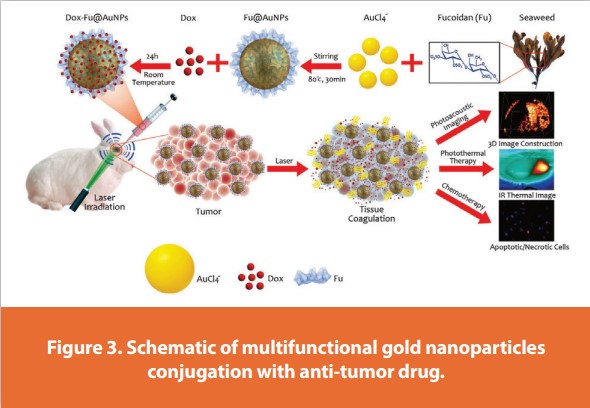
Kim et al. reported using Fu-coated gold NPs dosed with Dox to successfully kill choroidal tumors without recurrence two weeks after treatment administration. Fu was a great candidate to conjugate Dox with gold NPs as both are positively charged with Fu being negatively charged. Upon irradiation with a 0.11 W/cm2 laser, 100, 200, and 300 µg/ml gold NPs reached a max temperature of 48.6 ± 3.0, 56.8 ± 1.6, and 64.6 ± 1.7°C respectively. It was previously reported that temperatures in the range of 55–70°C can induce irreversible heat damage to tumors.45
In vitro, testing revealed the max cell viability achieved in the absence of irradiation using Dox-Fu gold NPs was 38% with a concentration of 300 µg/ml. With 2-minute laser irradiation, cell viability reached a peak low of 12% for Fu-Dox gold NPs at a concentration of 200 µg/ ml. Interestingly, irradiated Fu gold NPs without Dox resulted in almost no cell death. The researchers attributed this to the fact that Doxless Fu gold NPs only reached a temperature of 41 °C. However, the cytotoxic effects of the gold NPs cannot be fully attributed to Dox as the photothermal effect in cytotoxicity of tumor cells was significant. As discussed earlier, in the absence of laser irradiation, Dox-Fu gold NPs only result in a cell viability of 38% versus the 12% viability with laser treatment.
Similar results were observed in vivo (Figure 4A-D). Tumor size and progression was monitored in VX2 tumor-bearing rabbits for two weeks. The authors reported observing an increase in tumor size for rabbits injected with just Dox-Fu gold NPs without laser irradiation. The group that received both Dox-Fu gold NPs with laser treatment saw a decrease in tumor size up until day nine when the tumor began to disappear.
Imaging guidance can help to confirm the diagnosis and determine staging. Imaging modalities used to evaluate ocular tumors include orbital ultrasonography (US) or ultrasound biomicroscopy, computed tomography (CT), magnetic resonance imaging (MRI), and photoacoustic imaging (PAI).46,47,48
Kim et al. used photoacoustic (PA) imaging to visualize eye tumors (Figure 4E-F). To enhance the PA signals, the authors used gold NPs.
A big benefit of using gold NPs as an agent of drug delivery is its ability to also act as a contrast agent for visualization of the tumor. After injection of 100 µl of 200 µg/ml of Dox-Fu gold NPs, the contrast of the tumor was 2.6 times higher than the surrounding tissue. A 3D visualization of the tumor volume can also be constructed by combining a series of B-scan cross-sectional images, allowing us to clearly see the borders of the tumor.
Limitations and Future Clinical Applications
Pharmacotherapies for ocular diseases have shown significant promise in recent years. However, there remain several challenges that need further investigation, including the lack of effective treatments for some diseases, difficulty in developing drugs that can penetrate the eye's barriers, limited understanding of the underlying mechanisms of some diseases, and the high cost of treatments, which can make them inaccessible to some patients. In order to translate to clinical applications, there are several factors that need to be addressed, such as the development of more effective and specific treatments for specific ocular diseases, investigation of new drug delivery methods to improve treatment efficacy and reduce side effects, and continued investment in research to improve our understanding of ocular diseases and identify new targets for treatment. Recently, the gene therapy voretigene neparvovec-rzyl (Luxturna) from Spark Therapeutics has been launched as a novel treatment technique to treat patients with Leber congenital amaurosis but carries a very significant treatment cost for both eyes of more than $850,000. It would be beneficial to reduce treatment costs to increase the use of gene therapy and regenerative medicine.
Conclusions
In conclusion, advanced nanopharmacotherapies represent a promising strategy for the treatment of ocular diseases. These advanced techniques have been utilized to develop new and more effective therapeutics to deliver drugs to the eye. However, significant challenges still remain, including the need to overcome the barriers that prevent drugs from reaching their intended targets in the eye, and the need to improve our understanding of the underlying mechanisms of ocular diseases. Despite these challenges, results to date provide evidence that advances in ocular therapeutics advances in ocular therapeutics have the potential to revolutionize the treatment of ocular diseases and improve patient outcomes. Further research and investment in this area is therefore critical to ensure that the benefits of these advances are realized and that patients have access to the most effective treatments for their conditions.
Funding
This work was funded by grants from the National Eye Institute 1R01EY033000 (CHL and YMP), 1R41EY031219 (YMP), Fight for Sight-International Retinal Research Foundation (YMP: FFSGIA16002), Alcon Research Institute Young Investigator Grant (YMP), unrestricted departmental support from Research to Prevent Blindness, generous support of the Helmut F. Stern Career Development Professorship in Ophthalmology and Visual Sciences (YMP), and the University of Michigan Department of Ophthalmology and Visual Sciences. This research utilized the Core Center for Vision Research funded by the National Eye Institute (P30 EY007003).
References
- Burton, M. J. et al. The Lancet global health Commission on global eye health: vision beyond 2020. The Lancet Global Health 9, e489-e551 (2021).
- Solomon, S. D., Lindsley, K., Vedula, S. S., Krzystolik, M. G. & Hawkins, B. S. Anti‐vascular endothelial growth factor for neovascular age‐related macular degeneration. Cochrane Database of Systematic Reviews (2014).
- Mitchell, P., Liew, G., Gopinath, B. & Wong, T. Y. Age-related macular degeneration. The Lancet 392, 1147-1159 (2018).
- Matsumoto, H. et al. Quadrant laser photocoagulation trial to ameliorate choroidal congestion in central serous chorioretinopathy. Jpn. J. Ophthalmol., 1-8 (2023).
- Yeung, L., Lima, V. C., Garcia, P., Landa, G. & Rosen, R. B. Correlation between spectral domain optical coherence tomography findings and fluorescein angiography patterns in diabetic macular edema. Ophthalmology 116, 1158-1167 (2009).
- Vaz-Pereira, S. et al. Optical coherence tomography features of active and inactive retinal neovascularization in proliferative diabetic retinopathy. Retina 36, 1132-1142 (2016).
- Ishibazawa, A. et al. Characteristics of retinal neovascularization in proliferative diabetic retinopathy imaged by optical coherence tomography angiography. Invest. Ophthalmol. Vis. Sci. 57, 6247-6255 (2016).
- Kuehlewein, L. et al. Optical coherence tomography angiography of type 1 neovascularization in age-related macular degeneration. Am. J. Ophthalmol. 160, 739- 748. e732 (2015).
- Nguyen, V.-P. et al. Gold nanorod enhanced photoacoustic microscopy and optical coherence tomography of choroidal neovascularization. ACS Applied Materials & Interfaces 13, 40214-40228 (2021).
- Nguyen, V. P. et al. Contrast agent-enhanced multimodal photoacoustic microscopy and optical coherence tomography for imaging of rabbit choroidal and retinal vessels in vivo. Sci. Rep. 9, 1-17 (2019).
- Tian, C., Zhang, W., Nguyen, V. P., Wang, X. & Paulus, Y. M. Novel photoacoustic microscopy and optical coherence tomography dual-modality chorioretinal imaging in living rabbit eyes. JoVE (Journal of Visualized Experiments), e57135 (2018).
- Tian, C., Zhang, W., Mordovanakis, A., Wang, X. & Paulus, Y. M. Noninvasive chorioretinal imaging in living rabbits using integrated photoacoustic microscopy and optical coherence tomography. Opt. Express 25, 15947-15955 (2017).
- Zhang, W. et al. High-resolution, in vivo multimodal photoacoustic microscopy, optical coherence tomography, and fluorescence microscopy imaging of rabbit retinal neovascularization. Light: Science & Applications 7, 103 (2018).
- Nguyen, V. P., Li, Y., Zhang, W., Wang, X. & Paulus, Y. M. High-resolution multimodal photoacoustic microscopy and optical coherence tomography image-guided laser induced branch retinal vein occlusion in living rabbits. Sci. Rep. 9, 1-14 (2019).
- Nguyen, V. P., Li, Y., Zhang, W., Wang, X. & Paulus, Y. M. Multi-wavelength, en-face photoacoustic microscopy and optical coherence tomography imaging for early and selective detection of laser induced retinal vein occlusion. Biomedical optics express 9, 5915-5938 (2018).
- Nguyen, V. P. et al. In vivo 3D imaging of retinal neovascularization using multimodal photoacoustic microscopy and optical coherence tomography imaging. Journal of imaging 4, 150 (2018).
- Nguyen, V. P. et al. Chain-like gold nanoparticle clusters for multimodal photoacoustic microscopy and optical coherence tomography enhanced molecular imaging. Nature Communications 12, 34 (2021).
- Nguyen, V.-P. et al. Plasmonic gold nanostar-enhanced multimodal photoacoustic microscopy and optical coherence tomography molecular imaging to evaluate choroidal neovascularization. ACS sensors 5, 3070-3081 (2020).
- Chen, J. et al. Thin layer-protected gold nanoparticles for targeted multimodal imaging with photoacoustic and CT. Pharmaceuticals 14, 1075 (2021).
- Zhang, K., Zhang, L. & Weinreb, R. N. Ophthalmic drug discovery: novel targets and mechanisms for retinal diseases and glaucoma. Nature reviews Drug discovery 11, 541- 559 (2012).
- Reimondez-Troitiño, S., Csaba, N., Alonso, M. & De La Fuente, M. Nanotherapies for the treatment of ocular diseases. Eur. J. Pharm. Biopharm. 95, 279-293 (2015).
- Chow, E. K.-H. & Ho, D. Cancer nanomedicine: from drug delivery to imaging. Sci. Transl. Med. 5, 216rv214-216rv214 (2013).
- Weber, J., Beard, P. C. & Bohndiek, S. E. Contrast agents for molecular photoacoustic imaging. Nature methods 13, 639-650 (2016).
- Park, W. et al. Biodegradable silicon nanoneedles for ocular drug delivery. Science Advances 8, eabn1772 (2022).
- Sauvage, F. et al. Laser-induced nanobubbles safely ablate vitreous opacities in vivo. Nature nanotechnology 17, 552-559 (2022).
- Zahir-Jouzdani, F., Mottaghitalab, F., Dinarvand, M. & Atyabi, F. siRNA delivery for treatment of degenerative diseases, new hopes and challenges. Journal of Drug Delivery Science and Technology 45, 428-441 (2018).
- Esfandyarpour, R. et al. Label-free electronic probing of nucleic acids and proteins at the nanoscale using the nanoneedle biosensor. Biomicrofluidics 7, 044114 (2013).
- Maurizi, E. et al. Nanoneedles Induce Targeted siRNA Silencing of p16 in the Human Corneal Endothelium. Advanced Science 9, 2203257 (2022).
- Wu, Y. et al. Nanosuspension-loaded dissolving bilayer microneedles for hydrophobic drug delivery to the posterior segment of the eye. Biomaterials Advances 137, 212767 (2022).
- Yadav, K. S., Rajpurohit, R. & Sharma, S. Glaucoma: current treatment and impact of advanced drug delivery systems. Life Sci. 221, 362-376 (2019).
- Khandan, O., Kahook, M. Y. & Rao, M. P. Fenestrated microneedles for ocular drug delivery. Sensors and Actuators B: Chemical 223, 15-23 (2016).
- Kim, Y.-C., Park, J.-H. & Prausnitz, M. R. Microneedles for drug and vaccine delivery. Advanced drug delivery reviews 64, 1547-1568 (2012).
- Del Amo, E. M. & Urtti, A. Rabbit as an animal model for intravitreal pharmacokinetics: Clinical predictability and quality of the published data. Exp. Eye Res. 137, 111-124 (2015).
- Qin, Y. et al. Photo-mediated ultrasound therapy for the treatment of corneal neovascularization in rabbit eyes. Translational Vision Science & Technology 9, 16-16 (2020).
- Ucgul, R. K., Celebi, S., Yilmaz, N. S., Bukan, N. & Ucgul, A. Y. Intrastromal versus subconjunctival anti-VEGF agents for treatment of corneal neovascularization: a rabbit study. Eye 35, 3123-3130 (2021).
- Bakri, S. J., Snyder, M. R., Reid, J. M., Pulido, J. S. & Singh, R. J. Pharmacokinetics of intravitreal bevacizumab (Avastin). Ophthalmology 114, 855-859 (2007).
- Kim, Y. C., Edelhauser, H. F. & Prausnitz, M. R. Targeted delivery of antiglaucoma drugs to the supraciliary space using microneedles. Invest. Ophthalmol. Vis. Sci. 55, 7387-7397 (2014).
- Rao, A. A., Naheedy, J. H., Chen, J. Y.-Y., Robbins, S. L. & Ramkumar, H. L. A clinical update and radiologic review of pediatric orbital and ocular tumors. J. Oncol. 2013 (2013).
- Triozzi, P. L., Eng, C. & Singh, A. D. Targeted therapy for uveal melanoma. Cancer Treat. Rev. 34, 247-258 (2008).
- Nie, S., Xing, Y., Kim, G. J. & Simons, J. W. Nanotechnology applications in cancer. Annu. Rev. Biomed. Eng. 9, 257-288 (2007).
- Atashrazm, F., Lowenthal, R. M., Woods, G. M., Holloway, A. F. & Dickinson, J. L. Fucoidan and cancer: A multifunctional molecule with anti-tumor potential. Mar. Drugs 13, 2327- 2346 (2015).
- Li, B., Lu, F., Wei, X. & Zhao, R. Fucoidan: structure and bioactivity. Molecules 13, 1671-1695 (2008).
- Lu, K.-Y. et al. Development of a new type of multifunctional fucoidan-based nanoparticles for anticancer drug delivery. Carbohydrate polymers 165, 410-420 (2017).
- Tengdelius, M. et al. Synthesis and anticancer properties of fucoidan-mimetic glycopolymer coated gold nanoparticles. Chemical Communications 51, 8532-8535 (2015).
- Thomsen, S. Pathologic analysis of photothermal and photomechanical effects of laser–tissue interactions. Photochem. Photobiol. 53, 825-835 (1991).
- Kim, H. et al. Doxorubicin-fucoidan-gold nanoparticles composite for dual-chemo photothermal treatment on eye tumors. Oncotarget 8, 113719 (2017)
- de Graaf, P. et al. Guidelines for imaging retinoblastoma: imaging principles and MRI standardization. Pediatr. Radiol. 42, 2-14 (2012).
- Aerts, I. et al. Retinoblastoma. Orphanet J. Rare Dis. 1, 1-11 (2006).
Publication Detail
This article appeared in American Pharmaceutical Review:
Vol. 26, No. 6
Sep/Oct 2023
Pages: 10-16
Subscribe to our e-Newsletters
Stay up to date with the latest news, articles, and events. Plus, get special
offers from American Pharmaceutical Review delivered to your inbox!
Sign up now!