Abstract
Polyols (aka polyhydroxy co-solvents aka osmolytes) such as sucrose have long been known to impart thermodynamic stability to proteins in solution. Recent advances in biotechnology and in the development of proteins for therapeutic applications has led to an increased interest in these neutral co-solvents (more than 60 % of mAb formulations currently in market have polyhydroxy alcohols as excipients). Despite widespread efforts (> 40 years), the exact mechanism of the modulation of the chemical potential of proteins by polyols is not well understood and hence as a consequence, scientists do not dare to evaluate co-solvents other than a few selected ones for use in protein formulations. Considering that co-solvents should have opposite effects on thermodynamic stability and solubility, and non ideality of high concentration protein solutions complicates the kinetic and/or thermodynamic stabilization effect provided by polyols, the choice of a suitable co-solvent for a formulation may not be straightforward. Present review is focused on analyzing the concepts developed over the years towards the mechanistic understanding of the impact of polyhydroxy co-solvents on the stability of proteins in solution including the discussion of the widely accepted theory of preferential exclusion. Additionally, the review details the present state of knowledge in regards to the correlations between the effects of co-solvents on the thermodynamic and kinetic stability of proteins. The overall goal is to summarize the key results and the prevalent theories such as to provide the protein formulator with a better understanding of the selection criterion for the cosolvent/s.
Introduction
Despite recent advances in biotechnology, successful development of protein therapeutics is not an easy task and several issues are encountered during the preparation and administration of immunoglobulins and like molecules. The problems are usually associated with the long term stability of proteins and manifest in the form of physical instabilities such as aggregation and chemical instabilities such as deamidation and oxidation [1,2]. Since the function of a protein is governed by the structure of the protein, a loss of the native form has often been shown to be the rate limiting step in the process of irreversible inactivation of proteins and in the process of aggregation [3]. It is in regards to their ability to provide conformational stability to proteins in solution that polyols (polyhydroxy co-solvents, sugars, osmolytes etc.) have been found to be of immense importance to the biopharmaceutical industry.
Introduction of polyols as excipients into the solvent medium has been shown to affect protein properties in varying ways. The general observations are that these additives prevent the loss of enzymatic activity, inhibit irreversible aggregation of macromolecules and protect proteins against thermal and chemical denaturation [4-6]. Polyols have been seen to accumulate in certain osmotically stressed and freeze resistant organisms as well as in the medulla of human kidney [7]. There have been several ideas as to how these polyhydroxy cosolvents exert their affects on proteins and this has given rise a number of concepts. Although, several related and unrelated theories have been put forward to describe the observed stabilization effects, only one has gained widespread acceptance. This theory focuses on the preferential exclusion effect arising from increased non-attractions between polyols and protein (Figure 1) [8-10]. Several factors have been attributed to be the cause of the observed exclusion. These include excluded volume effects [11], effect of polyols on the interfacial tension [12, 13], solvophobicity of polyols [14], and effect of polyols on water structure [15]. Irrespective of the concept and/or the theory, it is certain that the net stabilization and/or destabilization of proteins by polyols is defined by the extent and nature of the preferential interaction parameters
It is important to understand for a formulator that an increase in the thermodynamic or thermal stability of a protein by a polyol may not necessarily result in the kinetic/shelf or aggregation stability of the concerned molecule of interest. The ultimate advantage provided by the polyol is of course dependent on the mechanism of aggregation and the polyol may not be able to provide the desired effect if the structural or the conformational stability is not the rate limiting step in the process of aggregation. An additional factor to consider during the usage of polyols is the impact of polyol on the solubility of the protein of interest as an increase in the thermodynamic stability of the protein by polyol necessarily means that the thermodynamic solubility of the protein is decreased by the presence polyol. Although, it is anticipated from the exclusion theory that addition of polyols should result in a decrease in protein solubility [11], several of the commonly used polyols have been shown to increase protein solubility [16-18]. Little if any explanation has been provided by authors as to what is the cause of this increased solubility. Possible explanations may however be obtained from data present in literature. One explanation comes from the articles published by Bolen and co-workers [19]. These authors measured the transfer free energies of amino acids from water to sugar solutions and showed that the transfer of side chains of most amino acids from water to the osmolyte solution is favorable. Such a favorable interaction between polyols and proteins (polyol inclusion and not exclusion) could result in the observed increase in protein solubility.
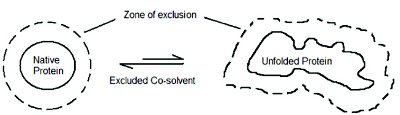
Figure 1. Schematic representation of the stabilization of protein in co-solvent solutions. Equilibiria is shifted to the native state [11].
It is imperative the effect of polyols on the thermodynamic and kinetic stability, and solubility of proteins be examined in detail before a decision on a suitable polyol is made. In this regards, the following discussion has been separated into sections on preferential interactions, kinetic verses thermodynamic stability and thermodynamic solubility. Finally, the last section provides a personal viewpoint on as to how exclusion and enhanced solubility could both be achieved simultaneously. A brief summary is provided at the end.
Preferential Interactions
When a protein is submerged in a solution containing both water and the cosolvent, interactions between protein and water, and protein and cosolvent govern the distribution of water and the cosolvent around the protein and in the bulk solution. These interactions between protein, cosolvent and solvent are conveniently described in terms of the preferential interaction parameter and the preferential interaction coefficient [11]. Preferential interaction parameter (Φ) describes the change in the chemical potential of the protein when the cosolvent concentration is increased by a small amount.

Where, m3 and m2 are the molal concentrations of cosolvent and protein respectively, T is the absolute temperature, P is pressure and μ2 is the chemical potential of protein using the convention of Scatchard [20] and Stockmayer [21] that component 1 denotes water, component 2 denotes protein and component 3 denotes cosolvent. Preferential interaction coefficient (Г) describes the amount of the cosolvent that must be added or removed from the bulk solvent when the protein concentration is increased by a small amount (in order to keep the chemical potential of the cosolvent constant).

From the measurement of preferential interaction coefficients and preferential interaction parameters, quantitative information can be obtained about protein-water and protein-cosolvent interactions. A negative value of the preferential interaction coefficient indicates that the protein-cosolvent interaction is unfavorable and cosolvent is excluded from the immediate vicinity of the protein molecules (i.e. the cosolvent molecules are present in higher concentration in the bulk solution relative to their concentration around the protein domain). A necessary consequence of the preferential exclusion of cosolvent from protein domain is the preferential hydration of protein molecules. A positive value of the preferential interaction parameter on the other hand indicates that cosolvent-protein interactions are favorable and protein surface is deficient in water.
Preferential interaction parameters and coefficients have been measured for a variety of protein-cosolvent combinations [4-8, 10, 22-25]. Table 1 summarizes a compilation of these parameters for ribonuclease under a variety of solution conditions. This table shows that polyols in general are excluded from protein domain. Additionally, it is also clear from the table that the extent of exclusion (or preferential hydration) depends on factors such as protein type, cosolvent type, cosolvent concentration, pH and temperature.
Once the preferential interaction parameter has been obtained, transfer free energy of the protein from pure solvent to cosolvent solution can easily be calculated by utilizing the following relation [11].

The above equation can be used to quantitate the effect of polyols on the chemical potential of the protein [25].
Although, thermodynamic experimental data clearly shows that osmolytes are excluded from protein domain, it does not provide any direct explanation of the molecular origin of the observed preferential interactions. It is important that the physiochemical basis of the origin of exclusion agrees with the observed dependence of the thermodynamic stability of proteins on the concentration and the type of the cosolvent. Some of the reasons for such a molecular exclusion will be discussed in the following sections.
Table 1. Selected examples of preferential interactions of polyols with globular ribonuclease (RNase)
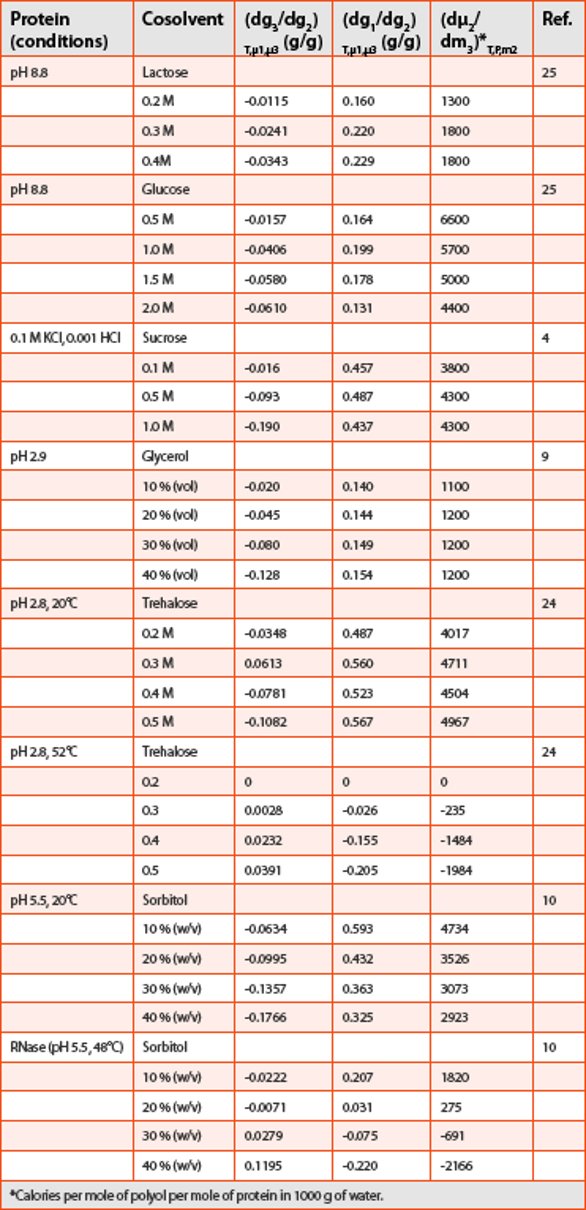
Surface Tension
Pioneering work by Gibbs showed that the concentration of solutes that increase the surface tension of water is lower at the air-water interface then in the bulk solution, while the concentration of solutes that decrease the surface tension of water is greater at the air-water interface then in the bulk solution [26]. Since addition of protein molecules to a solution results in the generation of protein-solvent interface (analogous to air-water interface), solutes that increase the surface tension of water are anticipated to be excluded from this interface. Such stabilization of macromolecules in water by the surface energy needed to create a cavity in the solvent before the introduction of the macromolecule was first proposed by Sinanoglu et al [27]. Lee et al. obtained a very good correlation between the increase in the surface tension of water by sucrose and its stabilization of proteins against thermal unfolding [4]. Similar results were also obtained by Kaushik et al. with several different polyols [13]. A community of authors now believe that preferential hydration of proteins by polyols is simply a consequence of the cohesive force that is exerted by the cosolvents on water molecules. This cohesive force manifested by an increase in the surface energy of water results in an increase of the chemical potential of proteins and provides the observed thermodynamic stabilization.
Although, generally accepted, several observations contradict the surface tension theory. Glycerol, a linear polyhydroxy polyol which falls in the same class as sorbitol and mannitol, is known to decrease the surface tension of water [8, 9]. Yet it is a known stabilizer and is widely used for this purpose. Timasheff and co-workers have hypothesized that the reason for the exclusion of linear polyols such as glycerol lies in the solvophobicity of proteins towards the glycerol containing cosolvent solution. However, even if we consider this hypothesis as true, it becomes intriguing that the reason of exclusion of two closely related species of molecules, glucose and sorbitol should be entirely different. Inhibitions to accept such an inference made by Timasheff are supported by data available in literature. Sorbitol, which is also known as D-glucitol, and D-glucose impart nearly the same thermodynamic stability to proteins in solution [5]. Considering the similarity of the two molecules (sorbitol has hydroxyl while glucose has aldehyde group), it is easy to accept that both these molecules provide equivalent stabilization because of the similarity in the mechanisms of their action.
Water Structure
Another recurring hypothesis in the field of protein stabilization by polyols is that these solutes act by altering the structure of water [15, 28,29]. Two classes of solutes have been defined in this regards. First class of molecules such as sucrose and trehalose are referred to as kosmotropes (water structure makers or order makers) [30]. Second class of molecules such as urea are known as chaotropes (water structure breakers or disorder makers) [31]. As is obvious from the classification system, word kosmotropes and chaotropes originally were used to denote solutes that stabilized and destabilized respectively the structure of proteins and membranes in solution [30]. Lately however, these terms have more generally been used for solutes that increase or decrease the structuring of water.
Non ionic water structure makers such as TMAO and sucrose are very soluble solutes that enhance water structure via an increase in water-water hydrogen bonds, stronger hydrogen bonds and greater spatial ordering of water molecules [15]. Consequently, these molecules decrease the rate of water diffusion around the protein and decrease the exchange rate of backbone amide protons [32]. Additionally, because of their favorable interaction with water, these neutral molecules are solubilized in the bulk of the solution and are preferentially excluded from protein’s immediate domain. Greater structuring of water results in the dehydration of protein surface making the biomolecules less conformationally flexible and more stable. Another way to understand this concept is to take a look at what happens when protein unfolds. Protein unfolding accompanies the breaking of hydrogen bonds between amide groups in the protein interior. These broken hydrogen bonds have to be replaced by hydrogen bond formation between the now open amide groups, and water molecules. Such hydrogen bond exchange requires that some water-water hydrogen bonds are broken. This breaking of water-water hydrogen bonds becomes enthalpically unfavorable in the presence of the structure making solutes because of the reasons that have been mentioned above. Consequently, the macromolecule becomes thermodynamically stable in the presence of such solutes. Structure breakers on the other hand, breakdown the normal hydrogen bonding network of water. Exactly opposite to kosmotropes, chaotropes weaken the water-water hydrogen bonds and decrease the ordering of water molecules. Presence of water structure breakers thus results in greater conformational freedom for the macromolecule and drives the folded-unfolded equilibirium towards the unfolded state.
Warner proposed that the presence of equatorial hydroxyl groups in saccharides is the factor that is responsible for the observed effects of sugars on the structuring of water [33]. This is because the O-O distance between the equatorial hydroxyl groups on sugars coincides with O-O distance of the water lattice. Uedaira et al. found good correlation between the net stabilization effect and the number of equatorial hydroxyl groups [34]. Gekko et al. also observed that number of equatorial hydroxyl groups on the molecule plays a significant role in determining its stabilizing effect [35]. In yet another work, Uedaira et al. obtained the dynamic hydration numbers for a variety of polyols by measuring the spin lattice relaxation time using NMR [36]. The authors found that the increment in the thermal stability of proteins, equilibrium distribution coefficients of polyols and sugars in the denatured hemoglobin solution and the effect of sugars on the osmotic flow of water, all varied linearly with the dynamic hydration numbers of the polyhydroxy molecules.
Despite the presence of indirect evidences such as those mentioned above and the presence of weakly scattered reports in which authors have studied the effect of one or two of these polyhydroxy alcohols on the structure of water, no single report exists which shows the relative effect of several of these polyols on the structure of water and hence correlates this structuring effect with protein stability. Additionally, structure breakers such as urea have been shown to interact favorably with the side chains of most amino acids [19]. This favorable interaction between urea and protein groups clearly points towards a different mechanism for urea’s denaturing action. Moreover authors such as Batchelor et al. (who had utilized thermodynamic models to study the effect of denaturants and stabilizers on the structure of water) found no correlation between solutes ability to impact water structure and its ability to modulate protein stability [28].
Water Activity
Hydration has long been recognized as an important component of macromolecular structure, stability and dynamics [37]. Osmotically active polyols result in the generation of osmotic stress that can affect biochemical equilibrium by affecting the number of water molecules participating in a reaction [38]. Parsegian et al. have utilized basic thermodynamic equations to show that the two different views of molecular action, osmotic stress and preferential hydration, are complementary and that osmotic stress simply emphasizes the role of water in the process of solute exclusion [39]. According to these authors, if the macromolecule attracts water molecules more strongly then it attracts the solute molecules, it can be shown that the ratio of Nw/Ns (number of water molecules to number of solute molecules) in the vicinity of the macromolecule is bound to be higher then nw/ns (number of water molecules to number of solute molecules) in the bulk solution. Hence, we should expect to observe exclusion even for solutes such as sucrose that by nature may be inert towards the macromolecular surface. Miyawaki stressed on the importance of the relationship between the hydration of the folded and unfolded states of proteins, and the increase in the thermal stability of proteins by polyols [40]. By incorporating the effect of hydration state change of protein upon unfolding (Δn),the author showed that decrease in the activity of water (aw) by polyols can indeed result in the observed stabilization effects.
It is also important to note that the two concepts, osmotic stress and water structure, that are often used to explain the effect of polyols on the thermodynamic stability of proteins in solution are not mutually exclusive. For non ideal solutions (containing polyols), it has been shown that water activity can be approximated by the following equation [41].

Where, aw is the activity of water, Xs is the mole fraction of solute and the parameter α and β are calculated by fitting the equation to the experimental results. Experimental measurements have shown that water structure makers such as sucrose seems to give αw (activity coefficient of water) of smaller then 1 while structure breakers such as urea seems to give αw values of greater then 1 [41]. Parameter β, an indicator of the effect of polyols on the activity of water, has been shown to correlate very well with the parameters that have generally been used to describe structuredness of water [41].
Steric Exclusion
Steric exclusion effect arises because of the finite size of molecules and depends only on the number shape and size of the molecules involved [11,42]. When a protein molecule is introduced into a cosolvent solution, it is surrounded by both, water and cosolvent molecules. However, because of the difference in the size of water and cosolvent molecules, there is a region around the protein molecule wherein only the smaller water molecules can enter. As this region is devoid of cosolvent molecules, a concentration gradient exists between the bulk solution (away from the protein molecule) and the region immediately surrounding the protein molecule. This phenomenon of steric exclusion can easily be understood from Figure 2 [42]. The distance of closest approach for the water and cosolvent molecules to the protein surface depends on their respective radii. Evidently, the volume of the sphere from which cosolvent molecules are excluded is greater than the volume of sphere from which water molecules are excluded. Steric exclusion thus contributes unfavorably to the transfer of protein molecules from pure water to cosolvent solution because of the free energy required to maintain the concentration gradient for the cosolvent molecules. An essential consequence of the steric exclusion of the cosolvent from protein domain is the preferential hydration of protein molecules.
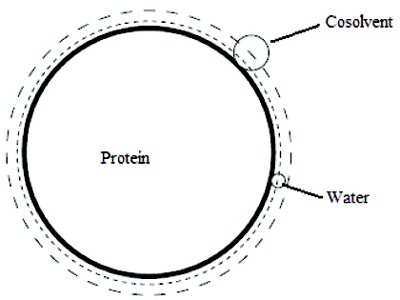
Figure 2. Excluded volume effect. Distance of closest approach of water or polyol molecule for the protein surface is determined by the radii of these molecules. Excluded volumes defined using water and osmolyte are the spheres shown by short dash and long dash respectively [11].
The magnitude of steric exclusion effect can be calculated using the scaled particle theory utilizing the changes in the configurational entropy for a mixture of hard spheres (water and cosolvent molecules) that is caused when a cavity of the size of protein molecule is introduced in the system [43]. This is a simple approach which takes into account the sizes of the solvent and the cosolvent molecules. Utilizing this approach, McClements calculated the contribution of steric exclusion to the transfer free energies of proteins from water to the cosolvent solution [43]. In this work, the contributions of differential interactions effects (effects that may arise because of other factors such as surface tension) was also calculated. Analysis of the relative magnitudes of the physiochemical contributions to the measured transfer free energies showed that the predominant contribution to the positive transfer free energies was indeed due to the steric exclusion of polyols. McClements has also done some numerical calculations of the impact of steric exclusion effect on the transfer free energy of a protein from water to a solution containing cosolvents of varying radii [11]. Simple calculations for the change in transfer free energy per unit surface area of protein per unit change in co-solvent concentration (dn/dc) for the case when the co-solvent concentration is expressed in weight percentage will show that on a weight to weight basis, co-solvent molecules with a molecular radius of 0.2 nm should be most effective in stabilizing proteins through a simple steric exclusion mechanism. This indeed is not the case and it is well recognized that cosolvent like sorbitol with a molecular radius of 0.33 nm are much more effective stabilizers of proteins than molecules such as glycerol (glycerol has a radius closer to 0.2 nm). The extent of role steric exclusion plays in the preferential hydration remains questionable not only because of the above mentioned discrepancy, but also because of the discrepancies observed in the field of protein precipitation by polyethylene glycols (PEGs). It is widely believed that steric exclusion is the mechanism by which PEGs increase the chemical potential of proteins and result in the precipitation of the bio-molecules out of the solution. However, simple theoretical calculations based on excluded volume considerations have shown that the predicted infl uence of PEG size on the precipitation of proteins does not correlate with experimental observations [44].
Solvophobicity
Hydrophobic effect [42] is believed to be the main force propelling proteins to fold in an aqueous environment and arises because of the unfavorable interaction of the non polar groups of proteins with water. Protein folding results in the burial of the hydrophobic groups, which in turn prevents the unfavorable interaction of the non polar groups with water. Solvophobicity is a similar term that has been introduced to describe the enhanced hatred (relative to water) of various groups on protein towards the cosolvent containing solution. This enhanced hatred results in the preferential exclusion of the solute molecules from protein surface and provides the observed stabilization effect. Several authors believe that molecules such as glycerol impart thermodynamic stabilization to proteins due to this solvophobic effect [14]. Unfavorable interaction of protein with the glycerol containing solvent drives the protein folded-unfolded equilibrium towards the folded state.
Polyol Hydrophobicity
It has been recognized that hydroxyl component of the polyhydroxy co-solvents is an important factor governing stabilization afforded to proteins. Despite the fact that protein folding is determined by hydrophobic interactions, the importance of the hydrophobicity of polyols especially those that have been shown to improve the solubility of small highly lipoohilic molecules has mostly gone unnoticed. The reason for putting polyol hydrophobicity in a section separate to that used to describe solvophobicity and surface tension is the opposite nature of the net result of the effect. Hydrophobic component on the polyols is anticipated to interact with the hydrophobic moieties of the protein molecules and hence would result in a net reduction of the thermodynamic stability of the protein opposite to the net stabilization that would be observed due to solvophobic (as defined) interactions and/or interactions which arise when such polyols are used that increase the surface tension of water (note that polyols that decrease the surface tension have not always been observed to decrease the thermal stability). Kumar et al. have recently shown that hydrophobicity index of the various sugars and polyols shows exceptional correlation with the stabilization afforded and hence stressed on the importance of the hydrophobicaly exposed component of the structure of poyols and sugars [45]. The data and explanation provided in this article clearly illustrates that such hydrophobic surface on polyols is an important determinant of the attractive interactions between polyols and hydrophobic protein surface.
Thermodynamic vs. Kinetic Stability
Protein aggregation is believed to be two step process [46]. In the first step, there is structural perturbation of the protein, whose thermodynamics are described by ΔGunf . Second step is the process of association dominated by intermolecular forces and reflected in the values of second virial coefficients. In theory, any of the two processes could be rate limiting. Clearly, the thermodynamic stabilization provided by polyhydroxy cosolvents would result in kinetic stabilization only if the first step is indeed the rate determining step in the process of aggregation. Addition of polyols to formulations wherein structure is not the guiding force for protein association may thus be a mere waste of time and effort. It is probably for the two step nature of the kinetics of aggregation that scientists often do not see a correlation between aggregation rate and conformational stability. At this time, it is also important to understand the role of ionic strength in the determination of this rate limiting step. High concentrations of salts and/or buffer concentrations minimize the repulsive electrostatic interactions observed at pH values away from the pI of the protein (for most mAbs wherein the pI’s are close to 9, the pH values of interest are 5-7). Minimization of the repulsive interactions by addition of salts may thus lead to a change in the rate determining step from second to first. For mAb solutions at pH 6, the amount of ionic strengths that have been observed to minimize such repulsive interactions is 15-20 mM [47]. Since most scientists work at ionic strengths that are usually higher than 20 mM, a correlation between the thermodynamic stability and shelf stability is often observed more times than it is not. Utility of sugars as excipeints in such high ionic strength solutions is thus obvious.
Solubility
Protein solubility is defined as the concentration of the soluble protein in equilibrium with its crystalline form in a given solvent under given solution conditions [48]. Solubility is determined by the net result of protein-protein, protein-solvent and solvent-solvent interactions. Because of the presence of varying functional groups, protein solubility becomes a complex function of a number of factors such as physical and chemical nature of the protein molecule and solution conditions (pH, temperature, dielectric constant etc). Since, addition of cosolvents affects the nature of net protein-solvent interactions; protein solubility is anticipated to be significantly affected by the presence of such additional solutes in the solution.
Effect of cosolutes on the solubility of proteins can be understood in terms of the concept of transfer free energy (transfer free energy is the change in the free of the system when the molecule in question is moved or transferred from solvent to the cosolvent solution). As shown earlier, transfer free energy of a protein from pure solvent to cosolvent solution can easily be calculated from the knowledge of the preferential interaction parameters. Additionally, the relationship between transfer free energy and protein solubility in the two solutions is given by the following equation [19]:
Here Cw and Ccs refers to the solubilities of the protein in the solvent and cosolvent solution and γw and γcs refers to the activity coefficients of protein in water and cosolvent solution respectively. Presence of cosolutes that bind to the protein (negative preferential interaction parameter/decrease in the chemical potential of the protein molecules) should result in an increase in protein solubility while presence of solutes that exclude from the protein domain should result in a decrease in the protein solubility (also see table 1 for the values of preferential interaction coefficients).
Although, several authors have utilized equilibrium dialysis experiments for the measurement of preferential protein-cosolvent interactions and for the calculation of the associated transfer free energies, little data is available in literature wherein authors have tried experimental methods to measure the solubilities of globular proteins in the presence and absence of polyols. Farnum et al. investigated the effect of glycerol on solubility of bovine pancreatic trypsin inhibitor (BPTI) with the background that glycerol preferentially hydrates protein molecules [16]. These authors observed that an increase in glycerol concentration increased the solubility of BPTI. Antipova et al. observed that presence of polyols such as sucrose resulted in an increase in the solubility of casein [17]. Similar effects of polyols have also been observed during the precipitation and crystallization of proteins by salts and/or polyethylene glycols. Lu et al. found that addition of glycerol decreased the amount of protein crystallized by high concentration of salts [49]. Paleg et al. have observed that presence of glycerol, sucrose or trehalose decreased the amount of glutamate synthetase precipitated by polyethylene glycols [50].
Experimental determination of the second virial coefficients (B22) of proteins in solution have also provided results that are in contradiction to that anticipated from the preferential exclusion theory. The thermodynamic parameter B22 appears in the virial expansion of the osmotic pressure term to represent non ideality in dilute colloidal solutions and is widely used to study the net protein-protein interactions in solutions. Measurements of B22 by different techniques such as light scattering and ultracentrifugation have shown that polyols in general decrease protein-protein attractive interactions in solution [51-52].
Pioneering work by Bolen and co-workers, wherein the authors measured solubilities of amino acids and their analogues in aqueous and cosolvent solutions, has given great insight about how specific groups on proteins interact with the osmolyte solution [19]. Side chains of most non polar amino acids were found to favor interaction with the osmolyte solution, indicating that presence of polyols would tend to expose the hydrophobic groups buried in protein interior. The major factor which overrides the side chain preference for the osmolyte solution and hence results in the stabilization of globular structure of proteins is the unfavorable interaction of the peptide backbone with the osmolyte solution. These results show that polyols should be anticipated to solubilize hydrophobic molecules better then water. A review of literature revealed that some of these polyols have indeed been shown to increase the solubility of small hydrophobic compounds [53, 54].
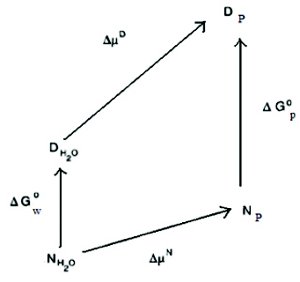
Figure 3. Thermodynamic box of stabilization of proteins by polyols. ΔG and Δμ represent change in the free energy or the chemical potential of the protein in going from one solvent to another. P = polyol, w = water, D = denatured state and N = native state.
Pioneering work by Bolen and co-workers, wherein the authors measured solubilities of amino acids and their analogues in aqueous and cosolvent solutions, has given great insight about how specific groups on proteins interact with the osmolyte solution [19]. Side chains of most non polar amino acids were found to favor interaction with the osmolyte solution, indicating that presence of polyols would tend to expose the hydrophobic groups buried in protein interior. The major factor which overrides the side chain preference for the osmolyte solution and hence results in the stabilization of globular structure of proteins is the unfavorable interaction of the peptide backbone with the osmolyte solution. These results show that polyols should be anticipated to solubilize hydrophobic molecules better then water. A review of literature revealed that some of these polyols have indeed been shown to increase the solubility of small hydrophobic compounds [53, 54].
Preferential Stabilization Yet Increased Stability
A typical thermodynamic box can be used to describe the observed exclusion and stabilization of proteins by polyols (Figure 3) [10]. This box adequately represents some general observations that have been made in regards to protein-polyol interactions. These include:
- Unfavorable interaction of the folded and the unfolded protein molecules with the cosolvent solution (transfer free energy of protein from water to cosolvent solution is positive).
- Interaction of the unfolded protein molecules with the cosolvent solution is more unfavorable then the interaction of the folded protein molecules with the cosolvent solution (higher energy barrier).
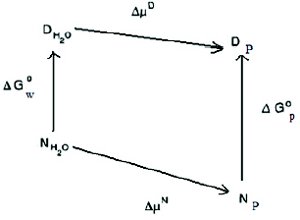
Figure 4. Thermodynamic box of stabilization of proteins by polyols. ΔG and Δμ represent change in the free energy or the chemical potential of the protein in going from one solvent to another. P = polyol, w = water, D = denatured state and N = native state.
Such a typical representation of the stabilization effect however does not explain the reported effects of polyols on the solubilities of proteins. An increase in the solubility of protein by polyol would require that the chemical potential of the protein is decreased by the presence of polyol. How do we explain stabilization and solubilization at the same time? For cases wherein an increase in protein solubility is observed, the thermodynamic box of stabilization can more appropriately be represented as shown in Figure 4. It should be noted that such a modified representation of the thermodynamic box is not entirely hypothetical and though rare, decrease of the chemical potential of proteins by polyols has been observed. An example of such a case has been reported by Xie et al [10].
Measurements of the preferential interaction parameters and preferential interaction coefficients have so far been restricted to proteins such as ribonuclease, chymotrypsin and chymotrypsinogen. Until data is generated for a wide variety of proteins that differ with respect to the nature of the exposed surface, it is difficult to speculate on the validity of the thermodynamic box that is shown in Figure 4. Additionally, it is also required that preferential interaction parameters and coefficients be calculated for a series of polyols that differ in their relative hydrophilicities. Coupled with the data from the experimental measurements of protein solubilities, results from the above mentioned studies would provide a good picture of the role of exclusion and/or binding in determining the effect of polyols on protein stability and solubility.
Summary
Modulation of the thermodynamic stability and solubility of the proteins by neutral polyols is a net result of two opposing forces: (1) Repulsive/neutral interactions determined by one or more of the several possible excluding forces (e.g. steric exclusion) and (2) Hydrophobic attractive interactions between the two species of molecules. Issues of solubility may become important especially for mAbs and like molecules that are usually required to be formulated at high concentrations. A formulator should thus keep in mind that it is possible to improve both, solubility and kinetic stability, with an appropriate choice of a polyol. Additionally, one should note that thermodynamic stabilization by polyols may not necessarily result in kinetic stabilization of the protein and an inappropriate unnecessary usage of a polyol could in fact be detrimental to the overall stability and hence real time stability studies should be conducted before a decision on the cosolvent is made. A platform development with sugars as excipients but without any sugar containing control is not recommended.
References
- M. C.Manning, K. Patel, R. T. Borchardt, Pharm. Res. 6, 903 (1989).
- T. Arakawa, S. Prestrelski, W. C. Kenney, J. F. Carpenter, Adv. Drug Del. Rev. 46, 307 (2001).
- S. J. Prestrelski, M. A. Pikal, T. Arakawa, Phar. Res. 12, 1250 (1995).
- L. C. Lee, S. N. Timasheff, J. Biol. Chem. 14, 7193 (1981).
- J. F. Back, D. Oakenfull, M. B. Smith, Biochemistry. 18, 5191 (1979).
- S. L.Bradbury, W. B. Jakoby, Proc. Natl. Acad. Sci. 69, 2373 (1972).
- P.W . Hochachka, G. N. Somero, Am. J. Physiol. 251, R197 (1986).
- K. Gekko, S. N. Timasheff, Biochemitry. 20, 4677 (1981).
- K. Gekko, S. N. Timasheff, Biochemitry. 20, 4667 (1981).
- G. Xie, S. N. Timasheff, Protein Sci. 6, 211 (1997).
- J. D. McClements, Crt. Rev. Food Sci. Nutrition. 42, 417 (2002).
- T. Y. Lin TY, S. N. Timasheff, Protein Sci. 5, 372 (1996).
- J. K. Kaushik, R. Bhat, J. Phys. Chem. B. 102, 7058 (1998). 1
- R. Sousa, E. M. Lafer, Methods. 1, 50 (1990).
- Q. Zou, B. J. Bennion, V. Daggett, K. P. Murphy, J. Am. Chem. Soc. 124, 1192 (2002).
- M. Farnum, C. Zukoski, Biophy.s J. 76, 2716 (1999).
- A. S. Antipova, M. G. Semenova, Carbohydrates polymers. 28, 359 (1996).
- M. Conti, M. Galassi, A. Bossi, P. G. Righetti, J. Chromat. A. 757, 237 (1997).
- L. Yufeng, D. W. Bolen, Biochemistry. 34, 12884 (1995).
- G. Scatchard, J. Am. Chem. Soc. 68, 2315 (1946).
- W. H. Stockmayer, J. Chem. Phys. 18, 58 (1950).
- J. C. Lee, K. Gekko, S. N. Timasheff, Methods Enzymol. 114, 49 (1985).
- K. Gekko, T. Morikawa, J. Biochem. 90, 39 (1981).
- G. Xie, S. N. Timasheff, Biophysical Chem. 64, 25 (1997).
- T. Arakawa, S. N. Timasheff, Biochemistry. 21, 6536 (1982).
- J. W. Gibbs, Trans. Conn. Acad. 3, 343 (1878).
- O. Sinanoglu, S. Abdulnur, Photochem. Photobiol. 3, 333 (1964).
- J. D. Batchelor, A. Olteanu, A. Tripathy, G. J. Pielak, J. Am. Chem. Soc. 126, 1958 (1958).
- T. H. Plumridge, R. D. Waigh, Pharm. Pharmacol. 54, 1155 (2002).
- P. M. Wiggnins, Cell. Mol. Biol. 47, 735 (2001).
- J. R. D. Xammer, J. Biol. Phys. 27, 73 (2001).
- I. Yu, M. Nagaoka, Chem. Phys. Lett. 388, 316 (2004).
- D. T. Warner, Nature. 196, 1055 (1962).
- H. Uedaira, H. Uedaira, Bull. Chem. Soc. Jpn. 53, 2451 (1980).
- K. Gekko, S. Koga, J. Biochem. 94, 199 (1983).
- H. Uedaira, H. Uedaira, Cell. Mol. Biol. 47, 823 (2001).
- R. S. Preisler, H. H. Chen, M. F. Colombo, Y. Choe, B. J. Short, D. C. Rau, Biochemistry. 14400 (1995).
- H. O. Hammou, P. D. Plaza, J. M. Ruiz-Snchez, New J. Chem. 1, 1453 (1998).
- V. A. Parsegian, R. P. Rand, D. C. Rau, Proc. Natl. Acad. Sci. 97, 3987 (2000).
- O. Miyawaki, Biochim. Biophys. Acta. 1774, 928 (2007).
- O. Miyawaki, A. Saito, T. Matsuo, K. Nakamura, Biosci. Biotech. Biochem. 61, 466 (1997).
- D. W. Bolen, Methods. 34, 312 (2004).
- J. McClements, Food Hydrocolloids. 15, 355 (2001).
- A. H. Donald, K. C. Ingham, J. Biol. Chem. 256, 12108 (1981).
- V. Kumar, R. Chari, V. K. Sharma, D. S. Kalonia, Int. J. Pharm. 413, 19 (2011).
- E. Y. Chi, S. Krishanan, B. S. Kendrick, B. S. Chang, J. F. Carpeneter, J. W. Randolph, Protein Sci. 12, 903 (2001).
- V. Kumar, N. Dixit, W. Fraunhofer, Abbott Bioresearch Center, Unpublished data.
- T. Arakawa, S. N. Timasheff, Methods Enzymol. 114, 49 (1985).
- X. Lu, J. X. Wang, C. B. Ching, Prog. Cry. Growth Char. Mat. 1, 201 (2002).
- L. G. Paleg, G. R. Stewart, J. W. Bradbeer, Plant Physiol. 75, 974 (1984).
- J. J. Valenete, K. S. Verma, M. C. Manning, W. W. Wilson, C. S. Henry, Biophys J. 89, 4211 (2005).
- G. T. Weatherly, G. J. Pielak, Protein Sci. 10, 12 (2001).
- M. Bertau, G. Jorg, Bioorgan. Med. Chem. 12, 2973 (2004).
- S. Wastani, V.K. Sharma, T.W. Patapoff, D.S. Kalonia, J. Pharm Sci. 100, 3096 (2011).
Author Biographies
Vineet Kumar,Ph.D., is a Sr. Scientist II at Abbott. He earned his MS (2002) and PhD (2007) in Pharmaceutical Sciences from University of Connecticut. In his current role he is primarily focused on preformulation characterization and early stage formulation development of therapeutic biological drug candidates. He has been invited to present his work at international conferences and has several publications, patents and abstract publications to his credit.
Shubhadra N. Singh, Ph.D., is a postdoctoral fellow at University of Connecticut and has more than 7 years of experience in protein purification and development.
Professor Devendra S. Kalonia is a faculty member at University of Connecticut. Professor Kalonia has more than 20 years of experience and is a well-known expert in the area of protein formulations.