Since the early 1980s, biotechnology products have shaped the pharmaceutical industry. A large number of monoclonal antibodies and therapeutic proteins have been approved, delivering meaningful contributions to patients’ lives, and are anticipated to be the major growth driver for the industry in the upcoming years [1-5]. In 2012, the list of the top 20 best-selling drugs included 8 biologics [6] (see Table 1).
Table 1. Top 20 best-selling drugs in 2012 (modified from [6])
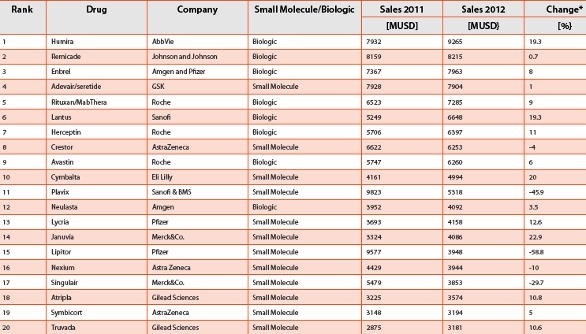
Mammalian cells are the expression systems of choice [7-10] due to their ability to properly fold and modify these complex proteins and antibodies. Tremendous process development efforts throughout the last decade have resulted in significantly increased manufacturing scales, product titers (up to 8g/L in fed batch processes), and recovery yields, thereby satisfying heightened market demand for existing and new products. Cell culture manufacturing scales up to 25 m3 operated in a batch, repeated batch, or fed batch mode, followed by a sequence of chromatography, filtration, and concentration steps delivering API batch sizes of up to 50 to 100 kg of protein/antibody, represent state of the art technology – a typical antibody production process is shown in Shukla and Thömmes 2010 [11-13]. Perfusion processes have been reported as an alternative manufacturing strategy to traditional large-scale fed-batch processes. Perfusion processes typically utilize smaller bioreactors in scales up to 1000 L, with high perfusion rates and process durations up to 200 days. [12, 14-23]
Since the generation of murine monoclonal antibodies via hybridoma technology by Kohler and Milstein [24], numerous milestones in the industry have been achieved, and advances in research, development, and manufacturing have been described. These include fully humanized recombinant monoclonal antibodies and the exploitation of antibody fragments for favorable characteristics, as well as the introduction of novel scaffolds based on antibody technology. Recently, the glyco-engineering of antibodies and fusion proteins are exploiting favorable modifications of antibody binding sites, as well as the antibody Fc sequence for enhanced effector function. The first non-radioactive antibody drug conjugates (e.g. Adcetris®, Kadcyla®, Mylotarg®) have received approval and will enable more targeted therapies and – in conjunction with companion diagnostics – open the gate to personalized healthcare. The version “2.0” of future proteins and antibodies based on today’s products will be a “facelift” by manipulating molecular frameworks to further improve binding specificity and efficacy, reduce needed therapeutic dose, conjugation to immunoactive substances, bi-specific binding antibodies, cytokine coupled entities, etc. A major breakthrough comparable to the first antibody technology is still pending. It should be stated that recent progress has been made in developing antibodies which interact more directly with the immune system – up-regulating its behavior towards antigens (e.g. cancer cells), in what is termed immunotherapy.
For today and tomorrow’s molecular formats, the major challenges will be funding and increased success rates in R&D. Although there is clear growth and trend towards the use of biologics, the pharmaceutical industry still faces significant challenges. Patent expiry is one of these major threats for biologics developers and manufacturers, particularly given the high R&D costs associated with bringing these biologics to market. This “patent cliff ” is well known in the small molecules world, and its impact has been felt with several drugs. In upcoming years, several biologics will run out of patent protection, and several “biosimilar” or “biobetter” manufacturers will enter the arena. In addition, increasing safety requirements, shift of significant growth to emerging markets, restricted market access, increased development costs and declining R&D productivity will put more pressure on the value and supply chain of innovation-driven companies.
However, this pressure has been vital to the industry in terms of innovating both, process and product development. Maturation of technologies and processes, higher degrees of automation, and process robustness driven by science and process management has been seen over the last decade.
In process development, high throughput robotics for both upstream and downstream process development have been vital to screen and improve cell lines, media compositions, chromatography media, etc. Increased titer results are due to improved expression vectors and media optimization supported by the higher automation degree and decreased scale (e.g. micro titer plates and tube bioreactors), allowing broader screens.
The “omics” approaches, including transcriptomics, proteomics, and metabolomics, along with the sequencing of the CHO genome have provided tools and data to support and enhance these efforts. Most of the significant improvements to date, however, have been based on the more conventional cell line/media approaches, with “omics” approaches still in search of significant success stories.
Another advancement which has increased efficiencies and enabled rapid startup of new manufacturing operations was the development and implementation of disposable / single-use technologies early on in the development processes. Now, the first concepts are wellestablished in manufacturing processes (e.g., filters, containers, culture flasks, bioreactors, etc.). With increasing titers and smart integrations of mature technologies, even fully disposable facilities have been reported. However, this is not only due to the availability of disposable technologies. The significant improvements in product titers and downstream purification yields driven by resin capacity and process throughput have enabled a highly efficient and commercially viable manufacturing based on disposables. One commercial provider of custom contract manufacturing, development services, and optimized technologies reported titers in their XD technology (integrated perfusion cell culture and product concentration by UF) utilizing a continuous cell line of up to 25g/L in a fully disposable system. Titers in more common fed batch cultures are up to 8 g/L in disposable bioreactors up to 2m3. This means that one batch derived from fully disposable systems that can be installed and started up quickly and at reduced capital cost can deliver approximately 16 kg of product into purification in an extremely small footprint – who would have thought this possible 20 years ago?
Next to bacterial fermentation for “relatively simple” recombinant proteins or antibody fragments, the work horse of the industry is still Chinese Hamster Ovary (CHO) cells. Yeast strains producing high yields of recombinant proteins such as EPO, or specific cell lines like the aforementioned continuous human cell line producing recombinant antibodies have been reported. However, these new cell culturing systems appear unlikely to replace the current work horses of the industry, E. coli bacteria and CHO cells, based on their significant successes and regulatory experience and acceptance. Non-cell culturing systems, such as transgenic animals or plants, have yet to make a significant dent in the established preference for E. coli or CHO cells.
In the late 1990s, the elimination of animal-derived raw materials both upstream (serum, peptones removed to yield chemically-defined medium) and downstream (Veggie Protein A affinity resin) delivered meaningful reductions in the virus contamination risks to cell culture processing. Additional risk mitigation was delivered through the implementation of viral clearance barriers in manufacturing processes (High Temperature Short Time heat treatment, novel virus filters, UV inactivation, etc.).
To increase efficiencies and mitigate risks, most companies have developed and implemented platform bioprocess technologies, beginning with a host cell line and media platform translated into a manufacturing platform based on standardized bioreactor platforms (including their design) and standardized purification processes (2 or 3 chromatography steps followed by formulation and filtration). The partnership with major suppliers – as in other industries – has enabled significant gains in throughput and robustness of processes based on these platforms. The processing options gained through these collaborations help the industry accelerate development work, gain knowledge, use common validation principles, and mitigate risks for scale up and technology transfers.
Today’s biopharmaceutical value and supply chains have matured. Supply chain risk mitigation through applications of mature technologies has become more and more important, as can be seen via examples of recent process contaminations having significant impact on a company’s well-being. In this case, “old” technologies such as HTST (High Temperature Short Time) heat treatment and other technologies analogous to pasteurization go through revitalization. In general, pilot and large scale manufacturing facilities are equipped with high tech sensors, sophisticated data analysis systems, and are highly automated to support process monitoring and continuous improvement. Pursuit of the PAT and QbD initiatives require enhanced analytics and data management capabilities.
While the discussion to this point has been focused on the bioprocess, it is important to note that advances and trends in pharmaceutical R&D have driven improvements in the ability to formulate, manufacture, and deliver biological drug products. Increasing desire for subcutaneous delivery of protein products has driven development of stable high concentration (100-200 mg/mL) formulations. Such applications can trigger the need to address significantly increased product viscosities, which present challenges in delivering the product. These challenges have been met both by improved formulation components as well as through improved delivery devices. Significant growth has occurred in the use of pre-filled syringe devices that can better support targeted self-administered applications (such as for arthritis indications). Development of sophisticated auto-injectors have further improved dosing simplicity for self-administration. Combination products (more than one API) have also advanced, one interesting example being the use of hyaluronidase co-formulated to facilitate subcutaneous delivery for products previously administered only intravenously. Such innovation provided for improvement in patient convenience and reduction of infusion reactions while enabling self-administration. On the protein drug product manufacturing front, manufacturers have adopted greater use of vapor phase hydrogen peroxide as a means of providing greater sterility assurance for these products which cannot be terminally sterilized.
Finally, the role of manufacturing has grown increasingly important. Manufacturing in the 21st century has to be agile, efficient and resilient. Planned and predictable performances along the supply chain are of crucial importance to guarantee uninterrupted supply to patients with high quality and acceptable financial performance. The goal for this part of the organization is to adapt to a changing portfolio of existing and new products, meeting quality and supply expectations and freeing up resources to be invested back into R&D – manufacturing has to become “externally supportive” and a strategic enabler for the industry [26-32].
What to Expect in the Next Decade
New molecular formats will be developed in pursuit of better answers to unmet medical needs. Combination products will be pursued as one means of improving patient convenience while reducing healthcare costs. Further increases in automation capability and efficiency of paperless systems for manufacturing will also be key to reducing costs and improving efficiencies. Two types of biopharmaceutical manufacturing facilities will likely be common – platform-based large scale manufacturing facilities in large-market regions, and small regional disposable plants in emerging markets. Given that large-scale (> 10 m3) plants have the ability to produce great quantities of proteins for blockbuster markets, and that more targeted (and smaller) patient populations can be addressed through moderate-scale (up to 2 m3) disposable systems, cell culture titers will not necessarily need to increase from current productivities. Resources will be spent more on improving control of protein product quality attributes to address Quality by Design and Process Analytical Technologies objectives and increasing downstream processing efficiency to drive costs down further. With increased product titers to a large extent based on increased cell culture growth, the physical characterization of bioreactors regarding mass transfer (especially CO2), mixing, and shear forces becomes more important [33-36]. A renewed focus on traditional engineering principles will be necessary. This will also be important to minimize risks for technology transfers and troubleshoot during technology transfers and process scale-up projects [37]. Expertise in modeling unit operations by Computational Fluid Dynamics and M3C (modeling, monitoring, measurement, and control) of bioprocesses via advanced approaches such as Artificial Neural Networks and Statistical Online Control will be relevant to supporting the next stage of process robustness and reliability [38-40].
Continuous processing trends should be noted. However, to date, only molecules requiring small residence time due to impact on product quality (e.g., Factor VIII, Epoitin, Factor VII, etc.) and specific expression systems have been established in commercial manufacturing processes. Recently, perfusion application in seed train for intermediate storage of large volume starter cultures and increase inoculation cell densities in production scale bioreactors to shorten operation time (subsequently leading to higher facility outputs) have been reported [41-43].
Much progress has been made in establishing reliable supply chains capable of delivering biopharmaceuticals for increasing applications that include numerous oncology and rheumatologic indications. The industry has matured significantly. While much has been achieved, unanswered questions remain. Pressures on the industry, such as the need for new molecular formats in the pipeline, evolving regulatory requirements driven by a desire to benefit from Quality by Design approaches and –most importantly – the need for global access to high quality bio-therapeutics addressing unmet medical needs at competitive costs, will drive the next decade’s achievements.
Author Biographies
Dr. Michael Pohlscheidt is Director of Manufacturing Operations at Genentech, Inc., Oceanside, CA. He received his degree in bioengineering in 2001 from the University of Applied Sciences in Aachen, Germany. His Ph.D. thesis was performed at Bayer HealthCare AG and Bayer Technology Services and guided by the University of Magdeburg, Germany (2005). From 2005 to 2010, he worked in different positions at Pharma Biotech Production & Development, Roche Diagnostics GmbH, Penzberg, Germany.
Dr. Robert Kiss is a Distinguished Engineer and the Director of Late Stage Cell Culture at Genentech, South San Francisco. He has worked in the process development and technical support of cell culture and fermentation processes for more than twenty years in the biopharmaceutical industry. He is a Fellow of the American Institute of Medical & Biological Engineers, and is a licensed professional engineer.
References
- Reichert JM. Monoclonal antibodies in the clinic. Nat Biotechnol. 2001; 19:819–822.
- Reichert JM. Therapeutic monoclonal antibodies: Trends in development and approval in the US. Curr Opin Mol Ther. 2002; 4:110–118.
- Reichert JM, Pavolu A. Monoclonal antibodies market. Nat Rev Drug Discov. 2004; 3:383–384.
- Drapeau M, Sullivan F, Moniz Carpenter J. Special Report: Blockbuster Then and Now-Trends for Billion-Dollar Drugs. Spectrum Therapy Markets and Emerging Technologies. 2007; 12:1–39.
- Munos B. Lessons from 60 years of pharmaceutical innovation. Nature Rev. 2009; 8:959–968.
- Top 20 Best Selling – Drugs of 2012. Genetic Engineering News. Insights and Intelligence. March 2013. Online: http://www.genengnews.com/insight-and-intelligence/top-20-bestselling- drugs-of-2012/77899775/?page=1
- Xie L, Wang DIC. Integrated approaches to the design of media and feeding strategies for fed batch cultures of animal cells. Trends Biotechnol. 1997; 15(3):109–13.
- Qi HN, Goudar CT, Michaels JD, Henzler HJ, Jovanovic GN, Konstantinov KB. Experimental and theoretical analysis of tubular membrane. Biotechnol Prog. 2003; 19(4):1183–1189.
- Wurm FM. Production of recombinant protein therapeutics in cultivated mammalian cells. Nat Biotechnol. 2004; 22:1393–1398.
- Sethuramann N, Stadheim TA. Challenges in therapeutic glycoprotein production. Curr Opin Biotechnol. 2006; 17(4):341–346.
- Heath C, Kiss R. Cell Culture Process Development: Advances in Process Engineering. Biotechnol Prog. 2007; 23:46–51.
- Su WW. Bioreactors, Perfusion. Encyclopedia of Cell technology. 2003; 978 – 993. John Wiley & Sons. Inc.
- Shukla A, Thömmes J. Recent Advances in Large-Scale Production of Monoclonal Antibodies and Related Proteins. Trends in Biotechnol. 2010; 28:253–261.
- Kompala D, Ozturk S. Optimization of high density perfusion bioreactors. In: Oztuk S, Hu W-S, editors. Cell culture technology for pharmaceutical and cellular therapies. New York: Taylor and Francis. 2006; 349–416.
- Voisard D, Meuwly F, Ruffieux PA, Baer G, Kadouri A. Potential of Cell Retention Techniques for Large-Scale High-Density Perfusion Culture of Suspended Mammalian Cells. Biotechnol & Bioeng. 2003; 82 (7):751–765.
- Ryll T, Dutina G, Reyes A, Gunson J, Krummen L, Etcheverry T. Performance of Small Scale CHO Perfusion Cultures Using an Cell Filtration Device for Cell Retention: Characterization of Separation Efficiency and Impact of Perfusion on Product Quality. Biotechnol & Bioeng. 2000; 69(4):440–449.
- Woodside SM, Boweb BD, Piret JM. Mammalian Cell Retention Devices For Stirred Perfusion Bioreactors. Cytotechnol. 1998; 28:163–175.
- Casthilo RC, Medrohno RA. Cell Retention Devices for Suspended- Cell Perfusion Cultures. In: Scheper T, Editor. Adv Biochem Eng Biotechnol. 2004; 74:129–169.
- Griffiths JB. Animal cell culture processes — batch or continuous? J Biotechnol. 1992; 22:21–30.
- Wang Z, Tan W, Poptic EJ, Belovich J. Feasibility of using a small-scale perfusion bioreactor with a novel cell retention device to inoculate a large-scale bioreactor. Conference Presentation at the American Chemical Society National Meeting, San Francisco, CA.
- Chu L, Robinson DK. Industrial Choices for protein production by large-scale cell culture. Curr Opin Biotechnol. 2001; 12(2):180–187.
- Henzler HJ. Kontinuierliche Fermentationmit tierischen Zellen - Teil 1: Aspekte der kontinuierlichen Prozessführung. Chemie Ingenieur Technik. 2012; 84 (9): 1469–1481.
- Henzler HJ. Kontinuierliche Fermentationmit tierischen Zellen - Teil 2: Techniken undMethoden der Zellrückhaltung. Chemie Ingenieur Technik 2012; 84 (9):1482–1496.
- Kohler, G, Milstein, C. Continuous Cultures of Fused Cells Secreting Antibody or Predefined Specificity. Nature, 256, 495-497, 1975.
- Correa HL. Agile Manufacturing as the 21st Century for Improving Manufacturing Competitiveness. In A. Gunasekaran, Agile Manufacturing as the 21st Century for Competitive Strategy. 2001; 3-24. Kidlington, Oxford, UK: Elsevier Science Ltd.
- Cremer P, Loesch M, Schrader U. Maximizing efficiency in pharma operations. The McKinsey Quarterly - Operations Practice. 2009 April:2-4.
- D´souza A, Keeling DJ, Phillips RD. Improving Quality in pharma manufacturing. The McKinsey Quarterly - Health CareStrategy. 2007: 1-7
- D´souza A, Keeling DJ, Phillips RD. Improving Quality in pharma manufacturing. The McKinsey Quarterly - Health CareStrategy. 2007
- Hayes RH, Schmenner RW. How Should You Organize Manufacturing? Harvard Business Review. 1978; 105-117. Harvard University, Boston, MA, USA
- Hayes RH, Wheelwright SC, Clark KB. Dynamic Manufacturing - Creating the Learning Organization. Free Press, New York, NY, USA 1988
- Hayes RH, Pisano G, Upton D, Wheelwright SC. Pursuing the Competitive Edge. Hoboken, NJ, USA: John Wiley & Sons. 2005.
- Wheelwright SC, Hayes RH. Competing Through Manufacturing. Harvard Business Review , 1985 2-12. Harvard University, Boston, MA,USA.
- Sieblist C, Ähle M, Pohlscheidt M, Jenzsch, M, Lübbert, A. Insights into Large Scale Cell Culture Reactors: I. Liquid Mixing and Oxygen Supply. Biotechnology Journal 2011; 6:1532-1546
- Sieblist C, Hägeholz O, Ähle M, Jenzsch M, Pohlscheidt M, Lübbert A. Insights into Large Scale Cell Culture Reactors: II. Gas-Phase Mixing and CO2-Stripping. Biotechnology Journal 2011;6:1547-1556
- Sieblist C, Jenzsch M, Pohlscheidt M, Lübbert A. Underlying principles Bioreactor Fluid Dynamics.in Comprehensive Biotechnology, second edition 2011, 6:517-530. In: Murray MooYoung (ed), Comprehensive Biotechnology, Second Edition, Volume 2; pp.47-62:Elsevier
- Schäpe S, Lübbert A, Pohlscheidt M, Sieblist C, Jenzsch M. Bioreactor Performance: Insights into Transport Properties of Aerated Stirred Tanks, American Pharmaceutical Review 2012; 15(6): 1-5
- Pohlscheidt M, Corrales M, Charanyia S, Fallon E, Bruch M, Jenzsch M, Sieblist C. Avoiding Pitfalls During Technology Transfer of Cell Culture Manufacturing Processes in the Pharmaceutical Industry – Mitigating Risk and Optimizing Performance. Pharmaceutical Outsourcing 2013;14(2):34-48
- Carrondo MJT, Alves PM, Carinhas N, Glassey J, Hesse F, Merten OW, Micheletti M, Noll T, Oliveira R, Reichl U, Staby A, Teixeira AP, Weichert H, Mandenius CF. How can measurement, monitoring, modeling and control advance cell culture in industrial biotechnology? Biotechnol. J. 2012, 7, 1522–1529
- Schäpe S, Kuprijanov A, Simutis R, Lübbert A, Pohlscheidt M, Jenzsch M. Batch-to-batch reproducibility of fermentation processes by robust operational design and control. Pharmaceutical Bioprocessing 2013;1(3):297-307
- Pohlscheidt M, Charaniya S, Bork C, Jenzsch M, Nötzel T, Lübbert A. Bioprocess and Fermentation Monitoring, in: The Encyclopedia of Industrial Biotechnology, Bioprocess, Bioseparation and Cell Technology. Editor Flickinger M. 2012; (2):147. John Wiley & Sons, Inc., ISBN 9780470054581
- Kloth C, Mac lsaaac G, Ghemrenariam H, Arunakumari A. Inoculum Expansion Methods, Recombinant Mammalian Cell Lines. Encyclopedia of Industrial Biotechnology: Bioprocess, Bioseparation and Cell Technology. 2010; 1–30. John Wiley & Sons. Inc.
- Heidemann R, Mered M, Wang DQ, Gardner B, Zhang C, Michaels J, Henzler HJ, Abbas N, Konstantinov K. A new seed-train expansion method for recombinant mammalian cell lines. Cytotechnology. 2002;38:99–108
- Pohlscheidt M, Jacobs M, Wolf S, Thiele J, Jockwer A, Gabelsberger J, Jenzsch M, Tebbe H, Burg J. Optimizing Capacity Utilization by Large Scale 3000 L Perfusion in Seed Train Bioreactors. Biotechnol Progress 2013;29(1):222-9