Introduction
Standard laboratory mouse models have limited utility in many types of experiments where biochemical or physiological processes are poorly reproduced due to species differences between mice and humans. One way to overcome this problem is to use mice that have been humanized for certain genes, cells, or tissues. Liver humanized mice have been used for a number of years to study infectious diseases that affect the human liver including hepatitis viruses and malaria parasite infections.1-4 These models are important for development and testing of treatments for these intractable infections because these human pathogens don’t infect mouse liver cells or their lifecycles are not adequately recapitulated in mice.5,6
Advances in the generation and maintenance of humanized mice have made them more robust tools and have stimulated interest in using them as models for answering additional liver-specific questions. For example, because mice and humans have significantly different pathways for drug metabolism and excretion by the liver, mice that have human liver enzymes are an improved test model for learning how new drugs or combinations will be metabolized and eliminated.4 In addition, with many new genomic editing therapies under development, liver humanized mice offer a unique testing environment for genomic editing therapy delivery methods, a significant challenge to implementation of these potentially transforming therapies.7,8 Finally, there is some evidence that these mice may eventually be appropriate models for other human metabolic diseases such as hypercholesterolemia, nonalcoholic fatty liver disease (NAFLD), and nonalcoholic steatohepatitis (NASH).9,10
Liver Humanized Mice
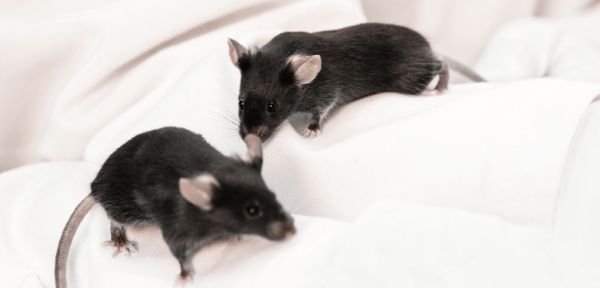
Liver humanized mice are immunodeficient mice in which liver injury has been induced by a genetic alteration in the mouse, creating damage to existing mouse liver cells and paving the way for engraftment of human liver cells to replace them. High-level repopulation (>90%) of human liver cells in the chimeric mice makes them an attractive model for liver-related research.9
The first liver humanized mouse was generated by overexpressing the urokinase-type plasminogen activator (uPA) in the immunocompromised SCID mouse to generate uPA-SCID mice. Overexpression of uPA leads to hepatotoxic effects and induces an optimal environment for engraftment with human hepatocytes.4 Repopulation rates associated with this method are sufficient for propagation and study of hepatotropic pathogens and human metabolism.11 However, due to their constitutive hepatotoxic overexpression of uPA starting in utero, these mice must be engrafted within two weeks after birth. This is very technically challenging and results in the loss of a large proportion of the transplanted animals. Less toxic, delayed, and inducible versions of this system have helped to alleviate some of these challenges, but result in lower repopulation levels.12 uPA-SCID mice also suffer from hypofertility, fragility, and a severe bleeding disorder, making their generation and maintenance challenging.11,13,14
The development of a liver humanized mouse based on knocking out (KO) an enzyme in the tyrosine catabolism pathway, fumaryl acetoacetate hydrolase (FAH), in an immunodeficient (Rag2-/-Il2rgnull) mouse has provided a more robust model, the liver humanized FRG® KO mouse, in which liver injury can be induced by withdrawal of a drug (2-(2-nitro-4-trifluoromethylbenzoyl) 1,3-cyclohexedione; NTBC) blocking the catabolic pathway at any time.15,16 These mice have consistently good levels of human liver cell engraftment and have been successfully infected with hepatitis B (HBV) and hepatitis C viruses (HCV).1,17 While these mice also require expert care and maintenance both before and after engraftment, they breed normally while maintained on NTBC and are more robust than their uPASCID counterparts.9 Rates of human liver cell engraftment generally correlate with levels of human serum albumin (1 mg/ml HSA ≈ 20% human hepatocytes), providing a way to monitor the degree of engraftment post-transplantation.9 Monitoring human albumin is also useful in infection experiments where decreasing human albumin levels over the course of infection may be used as a biomarker for the health of the hepatocyte graft. In addition, the FRG KO mouse, with its knockout of the FAH gene (rather than a transgene like the uPA mouse), cannot undergo genotype reversion so the human hepatocytes can be serially transplanted into other mice.9 This means that cells from a single human donor can be used in additional transplants to generate more mice with the same hepatocytes for a larger study or to maintain liver cells from a valuable donor after the lifespan of the initially transplanted mouse.9
Hepatotropic Pathogens
The utility of liver humanized mice has been demonstrated by their usefulness for research and drug development in hepatotropic pathogens. For example, liver humanized FRG KO mice have been shown to propagate both HBV and HCV (all genotypes) and both HBV- and HCV-infected mice respond to antiviral treatment.1,18,19 These mice remain healthy and the virus can be passaged using sera from one infected chimeric mouse to another with high titers similar to those inoculated with patient serum. These results show that liver humanized mice are a good model for HBV/HCV research and for evaluation of the efficacy of new antiviral drugs.1,20 Both uPA-SCID and FRG KO liver humanized mice have also been successfully infected with hepatitis E virus.21,22
Similarly, the liver stage of the causative agent of malaria, Plasmodium falciparum and other Plasmodium spp, can be detected in infected liver humanized uPA-SCID mice.23,24 This model has been used to show that antibodies against a molecule suspected to be involved in both HCV and P. falciparum invasion, CD81, can block infection, potentially identifying a new anti-malarial drug target.25,26
Drug Metabolism and Excretion
The liver plays a critical role in the metabolism and excretion of drugs and is a focus of research in this area. However, humans and mice exhibit many differences in terms of the metabolic enzymes that are present in liver cells and, therefore, significant differences in drug metabolism and excretion are often observed. While human cell lines and transgenic mice expressing human liver genes have been developed in attempts to address these problems, cell lines provide poor predictive value of human liver metabolic functions, and transgenic mice rely on the insertion of one or a few genes while metabolism is a complex process involving the action of many genes and pathways.9 These types of studies are critical as part of the portfolio of any new drug development program and it is common for animal models to fail to predict toxicity or metabolic behavior in humans. These disparities can cost time and money and even derail the approval process altogether. More accurate drug metabolism, elimination, and toxicity data provided at the animal testing stage by liver humanized mice could potentially provide powerful data that will more predictably translate to human trials.
Liver humanized uPA-SCID and FRG KO mice have been shown to express human phase 1 and 2 metabolic genes and many of these have been shown to be expressed and induced at similar levels to human hepatocytes.11,13,15,27-29 Liver humanized mice also convert from a rodent-type elimination pathway to a human-like excretion pathway upon engraftment.30 These data suggest that these mice may be a more accurate tool for studying the metabolism and excretion of new drugs than human cell lines or other animal models.
It also may be possible to obtain useful pharmacokinetic (PK) data from humanized mice. A study in uPA-SCID mice showed that the metabolism of 13 model compounds that use phase 1 and/or phase 2 pathways showed good correlation between human and humanized mouse values. Although the values were only semiquantitative, these mice might also be useful for providing human liver cells for this type of testing.31
Genomic Editing Formulation
Current genome editing therapies, including meganucleases, zinc finger nucleases (ZFNs), transcription activator-like effector nucleases (TALENS), and clustered regularly interspaced short palindromic repeat-associated nucleases (CRISPR-Cas9), offer the ability to directly edit and potentially repair some of the 3000 human genetic mutations that are known to cause disease.32,33 However, while genome editing therapies offer great promise for curing disease in patients, safe and efficient delivery of the vector carrying the therapy to the nucleus of targeted cells remains a challenge.8 These vectors must be introduced into the patient, evade nucleases and the immune system without causing an immune response, target the appropriate cell, enter the cell, translocate to the nucleus, and deliver their cargo.33 This presents researchers with a complex chain of delivery tasks to complete before the therapeutic agent even gets to its target!
Currently, multiple types of delivery systems are being tested. These include viral vectors, such as adenovirus, adeno-associated virus (AAV), or lentivirus, and non-viral vectors, including various chemical configurations of lipid-based nanoparticles.7,34-36 Viral vectors can potentially overcome many of the difficulties in targeting the therapy to the right place but are complicated by their limited size capacity and the possibility of toxicity and serious adverse events including immunogenicity, mutagenicity, and carcinogenesis.36 Non-viral vectors offer the possibility to overcome immunogenicity and capacity issues and are also easier to synthesize. However, while they often perform well in in vitro and ex vivo settings, there is still work to be done to improve their efficiency in vivo, a critical step for translation to the clinic.8,33
It is clear that in vivo testing will need to be done to find safe and effective delivery systems for genome editing therapies and liver humanized mice may offer a valuable model for this application. Also, once these therapies and delivery systems are developed, translation of genome editing therapies to human clinical trials will require demonstration that the therapy is likely to be delivered efficiently in humans. However, it is clear there are differences in the efficiency of transfection and possibly also in safety of these delivery systems for specific therapies between humans and mice used for standard animal testing.7 Liver humanized mice offer a way to test different delivery doses and modalities for each treatment in a manner that minimizes species differences that may affect the final delivery of the product to humans.
Future Applications in Human Disease Research
There are also tantalizing indications that liver humanized mice may be a useful model for other important human diseases involving liver function such as cholesterol dyslipidemia, NAFLD, and NASH. For example, it has been demonstrated that liver humanized FRG KO mice exhibit a human-like lipid and bile acid profile, even with a normal diet, suggesting they might be a good model for atherosclerosis and cholesterol metabolism.10 Future experiments with high-fat or other types of diets may also provide insight into diseases where deposition of fat in the liver is an important early stage of disease progression (NAFLD, NASH).
Conclusion
As laboratories that maintain liver humanized mice have improved their ability to provide these unique animals in larger numbers and at lower costs, the applications for their use have expanded. In addition to their utility as models for hepatotropic infectious disease, liver humanized mice have unique qualities that make them suitable for use in screening the metabolic activities of new drugs, for testing genome editing therapy delivery systems, and for studies of human liver diseases. Careful planning and close communication with expert laboratories who manage the complex husbandry and maintenance of these animals can provide powerful data to translate drug discoveries to human trials.
Acknowledgements
The author would like to acknowledge the contribution of a medical writer, Sandy Field, PhD, to preparation of this manuscript.
References
- Bissig KD, Wieland SF, Tran P, et al. Human liver chimeric mice provide a model for hepatitis B and C virus infection and treatment. J Clin Invest. 2010; 120 (3):924-930.
- Vaughan AM, Mikolajczak SA, Wilson EM, et al. Complete Plasmodium falciparum liverstage development in liver-chimeric mice. J Clin Invest. 2012;122(10):3618-3628.
- Sayed IM, Verhoye L, Cocquerel L, et al. Study of hepatitis E virus infection of genotype 1 and 3 in mice with humanised liver. Gut. 2017;66(5): 920-929.
- Meuleman P, Libbrecht L, De Vos R, et al. Morphological and biochemical characterization of a human liver in a uPA-SCID mouse chimera. Hepatology. 2005; 41(4): 847-856.
- Vercauteren K, de Jong YP, Meuleman P. HCV animal models and liver disease. J Hepatol. 2014;61(1 Suppl): S26-S33.
- Kaushansky A, Mikolajczak SA, Vignali M, Kappe SH. Of men in mice: the success and promise of humanized mouse models for human malaria parasite infections. Cell Microbiol. 2014;16(5): 602-611.
- Vercauteren K, Hoffman BE, Zolotukhin I, et al. Superior In vivo Transduction of Human Hepatocytes Using Engineered AAV3 Capsid. Mol Ther. 2016;24(6):1042-1049.
- He ZY, Men K, Qin Z, Yang Y, Xu T, Wei YQ. Non-viral and viral delivery systems for CRISPRCas9 technology in the biomedical field. Sci China Life Sci. 2017;60(5):458-467.
- Strom SC, Davila J, Grompe M. Chimeric mice with humanized liver: tools for the study of drug metabolism, excretion, and toxicity. Methods Mol Biol. 2010;640:491-509.
- Ellis EC, Naugler WE, Parini P, et al. Mice with chimeric livers are an improved model for human lipoprotein metabolism. PLoS One. 2013;8(11):e78550.
- Tateno C, Yoshizane Y, Saito N, et al. Near completely humanized liver in mice shows humantype metabolic responses to drugs. Am J Pathol. 2004; 165(3):901-912.
- Suemizu H, Hasegawa M, Kawai K, et al. Establishment of a humanized model of liver using NOD/Shi-scid IL2Rg null mice. Biochem Biophys Res Commun. 2008; 377(1): 248-252.
- Katoh M, Matsui T, Nakajima M, et al. Expression of human cytochromes P450 in chimeric mice with humanized liver. Drug Metab Dispos. 2004; 32(12):1402-1410.
- Foquet L, Wilson EM, Verhoye L, et al. Successful Engraftment of Human Hepatocytes in uPA-SCID and FRG® KO Mice. Methods Mol Biol. 2017;1506: 117-130.
- Azuma H, Paulk N, Ranade A, et al. Robust expansion of human hepatocytes in Fah−/−/ Rag2−/−/ Il2rg−/− mice. Nat Biotechnol. 2007; 25(8):903-910.
- Bissig KD, Le TT, Woods NB, Verma IM. Repopulation of adult and neonatal mice with human hepatocytes: a chimeric animal model. Proc Natl Acad Sci USA. 2007; 104(51):20507-20511.
- de Jong YP, Rice CM, Ploss A. New horizons for studying human hepatotropic infections. J Clin Invest. 2010;120(3):650-653.
- Dandri M, Burda MR, Torok E, et al. Repopulation of mouse liver with human hepatocytes and in vivo infection with hepatitis B virus. Hepatology. 2001; 33(4):981-988.
- Mercer DF, Schiller DE, Elliott JF, et al. Hepatitis C virus replication in mice with chimeric human livers. Nat Med. 2001; 7 (8):927-933.
- Vausselin T, Séron K, Lavie M, et al. Identification of a New Benzimidazole Derivative as an Antiviral against Hepatitis C Virus. J Virol. 2016;90(19):8422-8434.
- Allweiss L, Gass S, Giersch K, et al. Human liver chimeric mice as a new model of chronic hepatitis E virus infection and preclinical drug evaluation. J Hepatol. 2016;64(5):1033- 1040.
- Sayed IM, Foquet L, Verhoye L, et al. Transmission of hepatitis E virus infection to humanliver chimeric FRG mice using patient plasma. Antiviral Res. 2017;141:150-154.
- Sacci JB Jr, Alam U, Douglas D, et al. Plasmodium falciparum infection and exoerythrocytic development in mice with chimeric human livers. Int J Parasitol.2006; 36(3):353-360.
- Foquet L, Hermsen CC, Van Gemert GJ, et al. Molecular detection and quantification of Plasmodium falciparum-infected human hepatocytes in chimeric immune-deficient mice. Malar J. 2013;12:430.
- Meuleman P, Hesselgesser J, Paulson M, et al. Anti-CD81 antibodies can prevent a hepatitis C virus infection in vivo. Hepatology. 2008;48(6): 1761-1768.
- Foquet L, Hermsen CC, Verhoye L, et al. Anti-CD81 but not anti-SR-BI blocks Plasmodium falciparum liver infection in a humanized mouse model. J Antimicrob Chemother. 2015;70(6):1784-1787.
- Katoh M, Matsui T, Okumura H, et al. Expression of human phase II enzymes in chimeric mice with humanized liver. Drug Metab Dispos. 2005; 33(9):1333-1340.
- Nishimura M, Yoshitsugu H, Yokoi T, et al. Evaluation of mRNA expression of human drug metabolizing enzymes and transporters in chimeric mouse with humanized liver. Xenobiotica.2005; 35(9):877-890.
- Yoshitsugu H, Nishimura M, Tateno C, et al. Evaluation of human CYP1A2 and CYP3A4 mRNA expression in hepatocytes from chimeric mice with humanized liver. Drug Metab Pharmacokinet.2006; 21(6):465-474.
- Okumura H, Katoh M, Sawada T, et al. Humanization of excretory pathway in chimeric mice with humanized liver. Toxicol Sci. 2007; 97(2):533-538.
- Sanoh S, Horiguchi A, Sugihara K, et al. Prediction of in vivo hepatic clearance and half-life of drug candidates in human using chimeric mice with humanized liver. Drug Metab Dispos. 2012;40(2):322-328.
- Cox DBT, Platt RJ, Zhang F. Therapeutic genome editing: prospects and challenges. Nat Med. 2015; 21(2): 121-131.
- Wang HX, Li M, Lee CM, et al. CRISPR/Cas9-Based Genome Editing for Disease Modeling and Therapy: Challenges and Opportunities for Nonviral Delivery. Chem Rev. 2017;117(15):9874-9906.
- Wang M, Glass ZA, Xu Q. Non-viral delivery of genome-editing nucleases for gene therapy. Gene Ther. 2017;24(3):144-150.
- Chen X, Gonçalves MA. Engineered Viruses as Genome Editing Devices. Mol Ther. 2016;24(3):447-457.
- Wang L, Li F, Dang L, et al. In Vivo Delivery Systems for Therapeutic Genome Editing. Int J Mol Sci. 2016;17(5): E626.
Author Biography
Lander Foquet is a study director at Seventh Wave Laboratories working with the FRG in vivo humanized liver mouse model from Yecuris. Dr. Foquet’s main interests are infectious diseases (viral hepatitis, malaria), gene delivery, and drug metabolism.
Dr. Foquet obtained his PhD at Ghent University where he studied a humanized mouse model for malaria. This was followed by postdoctoral research on a fellowship from the Belgian American Educational Foundation at the Center for Infectious Disease Research in Seattle where he continued his work on malaria.