Introduction
Therapeutic mAbs have a propensity to aggregate under a variety of environmental conditions, similar to proteins in biological system.1,2 Protein aggregation in biological systems was reported to correlate with age-related or neurodegenerative-related diseases such as Alzheimer’s and Parkinson’s disease.3 Whereas, therapeutic mAb aggregation, as one of the potential degradation pathways may trigger immunogenicity, lower potency and thus may affect product safety, quality and efficacy. 4
Aggregates present in mAb products can be complex, varying by origin, type and size. Aggregate type can be covalent or non-covalent, reducible or non-reducible, and visible or subvisible. Aggregate size ranges from a few nanometers to a few millimeters in diameter, requiring multiple analytical techniques to obtain a holistic picture of the aggregate profile.5,6 The aggregation pathways were proposed as through (1) unfolded intermediates; (2) protein self-association or chemical linkages; and (3) chemical degradations.2 Aggregation can occur at all the stages of mAb production, including cell culture process, downstream process, fill/finish,7 and storage.8
Significant progress has been made to improve our current understanding of therapeutic mAb aggregation in terms of mechanism, design and control, as summarized in several excellent reviews.2,9-11 However, aggregation remains one of the major challenges in the development and commercialization of mAb products, with underlying mechanisms not always well-understood. The factors that influence mAb aggregation may be broadly classified into two categories, e.g. extrinsic (environment, bioprocessing, sample handling) and intrinsic (protein sequence and structure) factors.2 This article is intended to review and discuss our latest understandings of the intrinsic and extrinsic factors that lead to protein aggregation.
Intrinsic Factors
The aggregation propensity of an antibody can be determined by its intrinsic properties such as primary sequence and structure.12 Protein folds into a functional conformation that represents the lowest free energy and thus most stable, and this conformation is referred to as a native conformation.13 Antibody at its native state has less tendency to aggregate partly due to buried hydrophobic surfaces upon proper folding.14,15 However, compared to smaller size proteins, antibodies are more prone to aggregation, due to their large hydrodynamic radii and surface areas, non-symmetrical hydrophobicity and charge distributions.16,17
It was reported that amino acid sequence motifs enriched with glutamine (Q)/asparagine (N), hydrophobic or aromatic residues are potential aggregation prone regions. Whereas, charged residues (Asp, Glu, Lys, Arg and His) are rare in such regions.18,19 In addition, β-sheet is viewed as an energetically-favorable secondary structure that may form the building block of protein aggregates, such as amyloidosis.20,21 The dependency of aggregation on charge, hydrophobicity and β-sheet propensities of an amino acid sequence has formed the basis of studies to predict the aggregation propensity of a protein sequence22-24 or to design an aggregation-resistant antibody.25,26 Interestingly, it was found that aggregation hotspots are commonly located within CDRs (as well as the Fc domains) of commercial antibodies, suggesting the same peptide loops that mediate antigen recognition also mediate antibody aggregation.27
The tertiary structure that antibodies adopt at any given environment is determined by the amino acid sequence through competing molecular-scale fold-favoring interactions (e.g. electrostatic attraction, hydrophobic interaction and hydrogen bonding), and unfoldingfavoring interactions (e.g. geometric hindrance, electrostatic repulsion).28 Post-translational modification of amino acid, single site mutation may potentially disrupt that balance and promote protein unfolding and subsequent aggregation. For example, methionine oxidation in an IgG1 Fc region was reported to lead to an altered secondary and tertiary structure assessed by circular dichroism and NMR, and higher aggregation propensity upon heat stress.29 Histidine of an antibody may be oxidized and form a covalently linked aggregate.30,31 Antibody containing unpaired cysteine may form reducible aggregate through intermolecular disulfide bond upon agitation.32 In addition, deamidation and glycation have also been suggested to promote aggregation.33,34 Most recently, protein carbonylation was reported to positively correlate to higher antibody aggregation burst rate.8 Extrinsic factors, such as environment, excipients, or chemicals, could indirectly influence antibody aggregation through inducing conformational change, or modification of amino acid, are discussed in the next section.
Extrinsic Factors
Extrinsic factors refer to the environment that the therapeutic mAb product are exposed to, or process conditions that therapeutic mAb product go through. Biomanufacturing of an antibody starts with cell culture process over a period of approximately two weeks under controlled process conditions and feed strategy. During this process, antibodies, like other recombinant proteins produced through recombinant DNA technology, is synthesized by ribosomes that localize on the outer surface of endoplasmic reticulum (ER) in the cytosol. Upon the completion of synthesis, the newly-synthesized polypeptides undergo appropriate folding and are assembled into a multimeric complex, and subsequently acquire their asparagine-linked glycans in ER compartment. The folded glycoprotein was transported to Golgi apparatus for further modification to obtain finished product before being transported to cell membrane and secreted to extracellular cell culture suspension. Antibodies produced in cell culture processes are subsequently purified during the downstream process, through a combination of chromatography to remove process-related or productrelated impurities, such as HCP, DNA, virus, antifoam, aggregate and degradant. The purified antibody requires ultrafiltration/diafiltration (UF/DF) to buffer exchange into formulation buffer and reach the target protein concentration prior to bottling or bagging.
Cell culture processes may impose more stresses on mAb than downstream process, formulation, drug product fill and finish.35 Cell culture processes needs to grow the protein expressing cells that require a temperature of approximately 37°C, neutral pH, sparging and stirring. This requirement limits the flexibility for adjusting conditions to maximize protein stability. The secreted mAb is also exposed to the nutrient/feed in cell culture suspensions that is required for cell growth or desirable product quality, with their impact on mAb aggregation not well-understood.
In contrast, the extrinsic factors that influence mAb aggregation during downstream, formulation, drug product fill and finish process are better understood, which include pH, ionic strength, buffer type/composition, ionic strength, temperature, protein concentration, mechanical stress, and interactions with surfaces.2,4,7 During these production stages, mAbs are placed in a buffered environment that offer flexibility for improving protein stability in those process conditions. For example, chemical chaperones, such as salts, urea, glycerol, sucrose, arginine and other amino acids, may be included in a protein A elution buffer to minimize protein aggregation during low pH proA elution and viral inactivation step.36 Surfactants, such as polysorbates, are frequently included in high concentration mAb formulation buffers to minimize protein concentration-dependent aggregation, or the risk of agitation-induced aggregate formation as they interfere with hydrophobic interactions at the gas/liquid interface.37 The extrinsic factors and strategies to reduce aggregation during these processes are summarized in a few excellent review articles,2,4,7,9 and therefore will not be discussed further here. Our understanding on the extrinsic factors that influence antibody aggregation in cell culture process continues to evolve, and will be the focus of further discussion.
Antibody aggregation could occur intracellularly during the protein expression and folding process. Antibody or proteins are usually retained in ER compartment until they have their correct conformation. Therefore, ER is regarded as an organelle providing a protein quality control function within the cell. Factors influencing proper folding of protein in ER could promote protein aggregation. For example, disulfide bond formation during folding in ER is catalyzed by enzymes and requires oxidative environment.38 The unpaired cysteine was reported to potentially form a reducible aggregate and affect long-term storage of antibody,17 and adding Cu2+ to the cell culture medium was reported to reduce the level of free thiol for an mAb.39 Overexpression of target protein may also cause protein aggregation in ER, which could be optimized by reducing gene dose during cell line development.40,41
For fed-batch cell culture process, the mAb produced over a typical 10-17 days culture stays in the bioreactor till harvest, which undergoes various extracellular stresses in the bioreactor and forms aggregates. As summarized in Table 1, the extrinsic factors that have been reported to induce antibody aggregation are temperature, pH, ionic strength, osmolality, dissolved oxygen, agitation, metals, and medium components.8,41-43 Conflicting impact on aggregation by pH, temperature, dissolved oxygen, osmolality was reported by different studies. These discrepancies are likely due to confounding factors from other parameters, such as different cell line, different prevailing protein aggregation mechanism, and intrinsic properties of antibody used in those studies.42 For example, lower cell culture temperature was applied as a process lever to reduce aggregation,44 which occurs primarily extracellularly for an mAb. In contrast, another study reported increased antibody aggregate formation in CHO cells when lowering culture temperature.41 In this case, the aggregation was found to occur primarily in ER as a result of increased copies of light and heavy chain transcripts coupled with limited ER machinery at lower temperature compared to those at higher temperature for this particular cell line.
Table 1. List of extrinsic factors reported to infl uence therapeutic mAb aggregation in cell culture processes.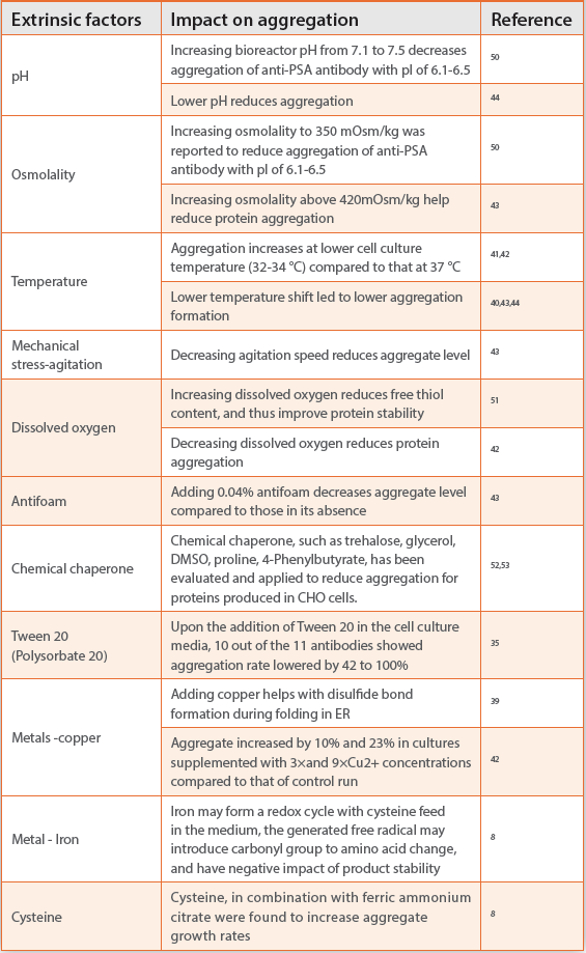
Trace metals are frequently used in cell culture medium to assure proper folding of the protein,39 achieve target N-glycan profiles,45 or function in the activation of enzymes and physiologically active substances inside the cell.46 Although trace metals were reported to induce protein oxidation in vitro,47 the impact of trace metal in cell culture media on antibody aggregation has not received much attention till recently.8 Ferric ammonium citrate and cysteine were reported as two major components in cell culture medium, contributing to increased level of protein carbonylation, which was reported to positively correlate to aggregate rates in antibody drug product.8 This result implies that ferric ions, cysteine, and possibly other metals and redox active components in cell culture may promote carbonylation-like modifications and subsequent aggregation. Protein carbonylation has been widely recognized and used as a universal marker of oxidative stress in living biological systems, and well documented in aging, agerelated degenerative, or neurodegenerative disorders diseases such as Alzheimer’s disease, Parkinson’s disease.48 Protein carbonylation, as a result of metal-catalyzed oxidation, was further confirmed in another in vitro stress study recently.49 Although many process levers have been applied to reduce protein aggregation as shown in Table 1, caution needs to be taken as any upstream changes may have potential impact on protein expression or quality.42 In addition, diff erent antibody may react diff erently to process relevant stress conditions, making it even harder to generalize and predict antibody behavior for any given stress condition.35 A design of experiment (DoE) approach is recommended to optimize cell culture process conditions to achieve desirable product quality of any given antibody expressed in a given cell line.43
Conclusion
Therapeutic mAbs have the propensity to aggregate under various stresses throughout all the stages of production. This review discussed the intrinsic and extrinsic factors that aff ect antibody aggregation during biomanufacturing process with the focus on cell culture process. Understanding the intrinsic factors inducing protein aggregation helps to engineer the antibody to be less prone to aggregation. Whereas, understanding the extrinsic factors of protein aggregation at each stage helps to develop a process or implement a process control strategy to minimize protein aggregation. Extrinsic factors infl uencing antibody aggregation in cell culture process are much more complex than those in the downstream, fi ll and fi nish processes. Some cell culture process condition changes were reported to result in the opposite outcome of aggregation. In addition, any upstream process condition change may also aff ect productivity, and other product quality. Therefore, a design of experiment approach is recommended to optimize cell culture process conditions to achieve desirable product quality of any given antibody expressed in a given cell line.
Acknowledgements
We are indebted to An Zhang and Ling Zhang for their valuable input and discussion.
References
- Maas C, Hermeling S, Bouma B, Jiskoot W, Gebbink MF. A role for protein misfolding in immunogenicity of biopharmaceuticals. J Biol Chem. 2007;282(4):2229-2236.
- Wang W, Nema S, Teagarden D. Protein aggregation--pathways and infl uencing factors. Int J Pharm. 2010;390(2):89-99.
- Kfoury N, Holmes BB, Jiang H, Holtzman DM, Diamond MI. Trans-cellular propagation of Tau aggregation by fi brillar species. J Biol Chem. 2012;287(23):19440-19451.
- Vazquez-Rey M, Lang DA. Aggregates in monoclonal antibody manufacturing processes. Biotechnol Bioeng. 2011;108(7):1494-1508.
- den Engelsman J, Garidel P, Smulders R, et al. Strategies for the assessment of protein aggregates in pharmaceutical biotech product development. Pharm Res. 2011;28(4):920- 933.
- Hamrang Z, Hussain M, Tingey K, et al. Characterisation of Stress-Induced Aggregate Size Distributions and Morphological Changes of a Bi-Specifi c Antibody Using Orthogonal Techniques. J Pharm Sci. 2015;104(8):2473-2481.
- Cromwell ME, Hilario E, Jacobson F. Protein aggregation and bioprocessing. AAPS J. 2006;8(3):E572-579.
- Purdie JL, Kowle RL, Langland AL, Patel CN, Ouyang A, Olson DJ. Cell culture media impact on drug product solution stability. Biotechnol Prog. 2016;32(4):998-1008.
- Wang W. Protein aggregation and its inhibition in biopharmaceutics. Int J Pharm. 2005;289(1- 2):1-30.
- Morris AM, Watzky MA, Finke RG. Protein aggregation kinetics, mechanism, and curve-fi tting: a review of the literature. Biochim Biophys Acta. 2009;1794(3):375-397.
- Roberts CJ. Therapeutic protein aggregation: mechanisms, design, and control. Trends Biotechnol. 2014;32(7):372-380.
- Wei Li PP, Weizao Chen1, Zhongyu Zhu, Yang Feng, and Dimiter S. Dimitrov. Antibody Aggregation: Insights from Sequence and Structure. antibodies. 2016;5(3):19-41.
- Anfi nsen CB. Principles that govern the folding of protein chains. Science. 1973;181(4096):223- 230.
- Dill KA. Dominant forces in protein folding. Biochemistry. 1990;29(31):7133-7155.
- DePristo MA, Weinreich DM, Hartl DL. Missense meanderings in sequence space: a biophysical view of protein evolution. Nat Rev Genet. 2005;6(9):678-687.
- Nishi H, Miyajima M, Nakagami H, Noda M, Uchiyama S, Fukui K. Phase separation of an IgG1 antibody solution under a low ionic strength condition. Pharm Res. 2010;27(7):1348-1360.
- Liu J, Nguyen MD, Andya JD, Shire SJ. Reversible self-association increases the viscosity of a concentrated monoclonal antibody in aqueous solution. J Pharm Sci. 2005;94(9):1928-1940.
- Wang X, Das TK, Singh SK, Kumar S. Potential aggregation prone regions in biotherapeutics: A survey of commercial monoclonal antibodies. MAbs. 2009;1(3):254-267.
- Sanchez de Groot N, Pallares I, Aviles FX, Vendrell J, Ventura S. Prediction of “hot spots” of aggregation in disease-linked polypeptides. BMC Struct Biol. 2005;5:18.
- Dobson CM. The structural basis of protein folding and its links with human disease. Philos Trans R Soc Lond B Biol Sci. 2001;356(1406):133-145.
- Chiti F, Taddei N, Baroni F, et al. Kinetic partitioning of protein folding and aggregation. Nat Struct Biol. 2002;9(2):137-143.
- Tartaglia GG, Pawar AP, Campioni S, Dobson CM, Chiti F, Vendruscolo M. Prediction of aggregation-prone regions in structured proteins. J Mol Biol. 2008;380(2):425-436.
- Fernandez-Escamilla AM, Rousseau F, Schymkowitz J, Serrano L. Prediction of sequencedependent and mutational effects on the aggregation of peptides and proteins. Nat Biotechnol. 2004;22(10):1302-1306.
- Buck PM, Kumar S, Wang X, Agrawal NJ, Trout BL, Singh SK. Computational methods to predict therapeutic protein aggregation. Methods Mol Biol. 2012;899:425-451.
- Lee CC, Perchiacca JM, Tessier PM. Toward aggregation-resistant antibodies by design. Trends Biotechnol. 2013;31(11):612-620.
- Schaefer JV, Pluckthun A. Engineering aggregation resistance in IgG by two independent mechanisms: lessons from comparison of Pichia pastoris and mammalian cell expression. J Mol Biol. 2012;417(4):309-335.
- Wang X, Singh SK, Kumar S. Potential aggregation-prone regions in complementaritydetermining regions of antibodies and their contribution towards antigen recognition: a computational analysis. Pharm Res. 2010;27(8):1512-1529.
- Creighton TE. Protein folding. Biochem J. 1990;270(1):1-16.
- Liu D, Ren D, Huang H, et al. Structure and stability changes of human IgG1 Fc as a consequence of methionine oxidation. Biochemistry. 2008;47(18):5088-5100.
- Liu M, Zhang Z, Cheetham J, Ren D, Zhou ZS. Discovery and characterization of a photooxidative histidine-histidine cross-link in IgG1 antibody utilizing (1)(8)O-labeling and mass spectrometry. Anal Chem. 2014;86(10):4940-4948.
- Xu CF, Chen Y, Yi L, et al. Discovery and Characterization of Histidine Oxidation Initiated Crosslinks in an IgG1 Monoclonal Antibody. Anal Chem. 2017;89(15):7915-7923.
- Huh JH, White, A. J. White, Brych, S R., Franey, H., Matsumura, H. The Identification of Free Cysteine Residues Within Antibodies a Potential Role for Free Cysteine Residues in Covalent Aggregation Because of Agitation Stress. J Pharm Sci. 2013;102(6):1701-1711.
- Manning MC, Chou DK, Murphy BM, Payne RW, Katayama DS. Stability of protein pharmaceuticals: an update. Pharm Res. 2010;27(4):544-575.
- Taghavi F, Habibi-Rezaei M, Amani M, Saboury AA, Moosavi-Movahedi AA. The status of glycation in protein aggregation. Int J Biol Macromol. 2017;100:67-74.
- Dengl S, Wehmer M, Hesse F, Lipsmeier F, Popp O, Lang K. Aggregation and chemical modification of monoclonal antibodies under upstream processing conditions. Pharm Res. 2013;30(5):1380-1399.
- Shukla AA, Gupta P, Han X. Protein aggregation kinetics during Protein A chromatography. Case study for an Fc fusion protein. J Chromatogr A. 2007;1171(1-2):22-28.
- Fesinmeyer RM, Hogan S, Saluja A, et al. Effect of ions on agitation- and temperature-induced aggregation reactions of antibodies. Pharm Res. 2009;26(4):903-913.
- Frand AR, Cuozzo JW, Kaiser CA. Pathways for protein disulphide bond formation. Trends Cell Biol. 2000;10(5):203-210.
- Chaderjian WB, Chin ET, Harris RJ, Etcheverry TM. Effect of copper sulfate on performance of a serum-free CHO cell culture process and the level of free thiol in the recombinant antibody expressed. Biotechnol Prog. 2005;21(2):550-553.
- Estes B, Hsu YR, Tam LT, Sheng J, Stevens J, Haldankar R. Uncovering methods for the prevention of protein aggregation and improvement of product quality in a transient expression system. Biotechnol Prog. 2015;31(1):258-267.
- Gomez N, Subramanian J, Ouyang J, et al. Culture temperature modulates aggregation of recombinant antibody in cho cells. Biotechnol Bioeng. 2012;109(1):125-136.
- Jing Y, Borys, M., Nayak, S., Egan, S., Qian, Y., Pan, Shih-Hsie, Li, Z. J. Identification of cell culture conditions to control protein aggregation of IgG fusion proteins expressed in Chinese hamster ovary cells. Process Biochemistry. 2012;47:69-75.
- Paul AJ, Handrick R, Ebert S, Hesse F. Identification of process conditions influencing protein aggregation in Chinese hamster ovary cell culture. Biotechnol Bioeng. 2017.
- Gomes J, Hiller, G.W. Use of low temperature and/or low ph in cell culture. US Patent Application # US20080269132. 2008.
- Patrick Hossler P, Racicot, C., and McDermott, S. Targeted Shifting of Protein Glycosylation Profiles in Mammalian Cell Culture through Media Supplementation of Cobalt. J Glycobio. 2014;3(1):1000108.
- Yao T, Asayama Y. Animal-cell culture media: History, characteristics, and current issues. Reprod Med Biol. 2017;16(2):99-117.
- Ji JA, Zhang B, Cheng W, Wang YJ. Methionine, tryptophan, and histidine oxidation in a model protein, PTH: mechanisms and stabilization. J Pharm Sci. 2009;98(12):4485-4500.
- Dalle-Donne I, Giustarini D, Colombo R, Rossi R, Milzani A. Protein carbonylation in human diseases. Trends Mol Med. 2003;9(4):169-176.
- Kryndushkin D, Rao VA. Comparative Effects of Metal-Catalyzed Oxidizing Systems on Carbonylation and Integrity of Therapeutic Proteins. Pharm Res. 2016;33(2):526-539.
- Franco R, Daniela G, Fabrizio M, Ilaria G, Detlev H. Influence of osmolarity and pH increase to achieve a reduction of monoclonal antibodies aggregates in a production process. Cytotechnology. 1999;29(1):11-25.
- Trexler-Schmidt M, Sargis S, Chiu J, et al. Identification and prevention of antibody disulfide bond reduction during cell culture manufacturing. Biotechnol Bioeng. 2010;106(3):452-461.
- Onitsuka M, Tatsuzawa M, Asano R, et al. Trehalose suppresses antibody aggregation during the culture of Chinese hamster ovary cells. J Biosci Bioeng. 2014;117(5):632-638.
- Hwang SJ, Jeon CJ, Cho SM, Lee GM, Yoon SK. Effect of chemical chaperone addition on production and aggregation of recombinant flag-tagged COMP-angiopoietin 1 in Chinese hamster ovary cells. Biotechnol Prog. 2011;27(2):587-591.
Author Biographies
Dr. Li Zang is an associate director overseeing the Protein Analytical Development department of Biogen. Her Ph.D. focused on development and application of highly sensitive liquid chromatography-mass spectrometry technology for breast cancer biomarker discovery. After joining Biogen in 2005, Li developed her expertise in biopharmaceutical development especially in detailed protein structure characterization using separation and mass spectrometry. She has participated in the development of a large category of biopharmaceutical programs at Biogen over the last 13 years, including monoclonal antibodies, receptor-Fc fusion proteins; blood coagulation factors, bi-specific antibodies, antibody-drug conjugates, biosimilar programs and small endogenous proteins.
Dr. Yunyu (Linda) Yi is a Sr. Scientist in Protein Analytical Development department of Biogen. She has developed her expertise in recombinant therapeutic protein characterization by electrophoresis, liquid chromatography, as well as LC-MS or LC-MS/MS over the last 13 years. She has participated drug development for monoclonal antibodies, blood coagulation factors, bi-specific antibodies, fusion proteins, protein vaccines, and biosimilars for preclinical and clinical programs.